1. INTRODUCTION
Malaria is a tropical infectious disease with a high mortality rate, caused by Plasmodium species. There were 249 million malaria cases worldwide in 2022, 5 million more than in 2021, significantly exceeding pre- COVID-19 number (World Health Organization, 2023). In the WHO’s Southeast Asia Region, almost 94% of all malaria-related deaths occurred in Indonesia (World Health Organization, 2023). According to the Indonesian Ministry of Health (2023), the number of malaria cases increased significantly to 3.3 million in 2022, a 64.6% increase compared to 2021. One of the obstacles to providing effective malaria therapy is that Plasmodium parasites can acquire resistance to antimalarial drugs. The preferred antimalarial drugs have changed several times, starting with chloroquine before shifting to sulfadoxine/pyrimethamine and artemisinin (Giacometti et al., 2021). The risk of emerging resistance can be minimized by using artemisinin-based combination therapy alongside primaquine or natural antimalarial bioactive substances (Huynh, 2016).
The Strychnos genus has been traditionally used to treat malaria in Asia and Africa. Strychnos species produce antimalarial substances (Philippe et al., 2005). Strychnosligustrina Blume syn. Strychnoslucida R. (Indonesia: Kayu ular/Bidara laut/Songga), a plant that grows abundantly in Indonesia, has been traditionally used as an antimalarial remedy. Indonesian traditional medicine has long utilized Strychnos plants to formulate treatments for malaria (Setiawan et al., 2014; Taek, 2023). Several studies have demonstrated the in vitro antimalarial activity of aqueous extracts of S.ligustrina wood (Familasari et al., 2023; Manurung et al., 2019), and these findings were confirmed in vivo (Cahyaningsih et al., 2022, 2023). The effective doses identified via in vivo antimalarial assays are safe for consumption, as demonstrated by subchronic toxicity (Sadiah et al., 2023) and acute oral toxicity tests (Khasanah et al., 2024). Consequently, there is potential to develop medications using the aqueous extract of S. ligustrina wood as a cost-effective active ingredient in antimalarial drugs.
Despite extensive research on the bioactive compounds and pharmacological potential of S. ligustrina, the antimalarial potential of different woody stem parts (heartwood, sapwood, and bark) has not been thoroughly investigated. Previous studies of S. ligustrina extract as an antimalarial did not separate the sapwood and heartwood parts, and did not use bark (Manurung et al., 2019; Syafii et al., 2016). Heartwood is the stem’s center and is usually darker than the outer sapwood. Sapwood is the outer part of the wood, and its cell tissue remains alive, transporting water and nutrients from the roots to the leaves. The bark is the outermost layer of the tree, serving as a protective barrier. Previous studies have revealed that various wood parts influence the phytochemical composition and biological activity of extracts (Aulia et al., 2022; Ella Nkogo et al., 2022; Miranda et al., 2017; Prayogo et al., 2023; Zalsabila et al., 2024). However, the compounds that can be used as standard antimalarial compounds for S. ligustrina wood have not yet been determined.
Molecular docking studies on compounds identified by liquid chromatography–tandem mass spectrometry (LC-MS/MS) can be performed to determine the profile of active compounds in S. ligustrina wood with potential antimalarial activity. These advanced analytical techniques enable the precise identification and quantification of bioactive constituents, providing valuable insights into their therapeutic potential and mechanisms of action. This research is critical in drug development, as it offers a cost-effective and efficient approach to screening natural products for antimalarial activity (Orozco et al., 2024). Therefore, this study aimed to determine the yields of heartwood, sapwood, and bark extracts of S. ligustrina, examine their in vitro antimalarial activity, and analyze the active compound profiles of these extracts using LC-MS/MS and in silico studies. This research is expected to contribute to the growing body of evidence supporting the use of plant-derived compounds against malaria, potentially leading to the discovery of new, affordable, and effective treatments.
2. MATERIALS and METHODS
S. ligustrina trees were obtained in Dompu, West Nusa Tenggara, Indonesia. This tree herbarium was identified by the Silviculture Laboratory of the Forestry Department, Mataram University, Mataram, Indonesia (voucher 23-2-2023). Woody stems (logs) were taken from three trees with 15–17-cm diameters. These stems were divided into bark, heartwood, and sapwood. The segments were processed into a fine powder (40–60 mesh) using a Willey mill. A test sample weighing ± 2 g was dried in an oven at 103 ± 2°C until a consistent dry weight was attained to determine the powder’s moisture content (Arinana et al., 2024).
The powdered heartwood, sapwood, and bark were subjected to maceration using water at a 1:10 (w/v) ratio of powder to solvent. The extraction was performed over three 24-h cycles. The resultant filtrates were combined using a vacuum evaporator at 50°C to obtain a dry extract. The extraction yield was then calculated.
Phytochemical compound analysis was conducted using an ultra-high-performance liquid chromatography system (Vanquish UHPLC) coupled with a Tandem Q Exactive Plus Orbitrap HRMS (Q Extractive Orbitrap MS/MS, Thermo Fisher Scientific, Waltham, MA, USA) and an AccucoreTM C18 column with dimensions of 100 × 2.1 mm and a particle size of 1.5 μm (Catalogue number: 27101-102130, Thermo Fisher Scientific). Each of the three types of extracts was dissolved using methanol LCMS Grade (Merck, Darmstadt, Germany), then filtered with PTFE membrane syringe filter type SYTF (mdi Membrane Technologies, Camp Hill, PA, USA). Gradient elution was performed over 50 minutes at a flow rate of 0.2 mL/min to separate the phytochemical compounds. H2O with 0.1% formic acid (A) and Acetonitrile with 0.1% formic acid (B) were used as mobile phases in the gradient elution system, with the following steps: 0–1 min (95% A and 5% B), 1–25 min (95%–5% A and 5%–95% B), 25–28 min (95% A and 5% B), and 28–30 min (95% A and 5% B). Positive electrospray ionization was employed as the ionization technique, and Q-Orbitrap was used as the mass analyzer. In both MS and MS/MS modes, the system was set to a range of 100–1,500 m/z with a resolution of 70,000 FWHM, a capillary temperature of 320°C, a column temperature of 30°C, and a spray voltage of (+) 3.8 kV. Additionally, an envelope gas flow rate of 15 mL/min and an auxiliary gas flow rate of 3 mL/min were used. Compound names were identified using the PubChem, COCONUT, and ChemSpider databases. The relative abundance of each compound was calculated by comparing its area percentage to the total area percentage of all compounds (Andianto et al., 2024).
The in vitro antimalarial activity assay followed the protocol of Manurung et al. (2019) and used the Plasmodium falciparum strain 3D7, which is sensitive to chloroquine. The extracts were prepared by dissolving 10 mg of the sample in 1 mL of dimethyl sulfoxide to create a stock solution. Then, serial dilutions were made from the stock solution to obtain concentrations of 1,000 μg/mL, 100 μg/mL, 10 μg/mL, 1 μg/mL, and 0.1 μg/mL. The parasites used in this test were synchronous (ring stage) with approximately 1% parasitemia (5% hematocrit). Two microliters of the test solution at various concentrations were taken and placed into each well of a 96-well plate. The, 198 μL of the parasite were added (each concentration was made in duplicate). The test well was then inserted into the chamber and exposed to a mixture of gases (5% O2, 5% CO2, and 90% N2). The chamber containing the test wells was incubated for 48 h at 37°C. The cultures were harvested, and thin blood films were made with 20% Giemsa stain.
Under a microscope, the number of infected erythrocytes per 1,000 normal erythrocytes was counted after data analysis on the generated blood smear. After that, the percent growth and inhibition were calculated using the data. The following equations were used to determine percent growth and inhibition, respectively:
To determine the IC50 (the concentration of the test material that inhibited the parasite’s growth by 50%), statistical analysis was performed using probit analysis based on the percent inhibition data. This analysis involved determining the relationship between the percent inhibition of the parasite and the extract concentrations.
The compounds of each extract identified based on LCMS were studied for their molecular binding mechanism to 5 target proteins (Table 1) in the treatment of malaria with the aim of killing the parasite. The selection of protein was limited to proteins that must come from P. falciparum 3D7, according to the one used at the in vitro stage. The crystal structure of proteins was obtained from the protein data bank (https://rcsb.org). Ligand preparation was accomplished by limiting the energy of the MM2 approach on the ligand’s molecular structure (Chem3D Pro 12.0, CambridgeSoft, Cambridge, MA, USA). AutoDockTools 1.5.6 (The Scripps Research Institute, San Diego, CA, USA) was used to prepare receptors by eliminating non-protein molecules, adding Kolman charges, and adding polar hydrogen. Docking method validation (Redocking) was performed by using the co-crystal ligands in each protein (less than 2 °C). Receptor–ligand binding was visualized using the Discovery Studio Visualizer v.21.1.0.20298 (Dassault Systemes, Vélizy-Villacoublay, France).
Protein (PDB ID) | Center coordinate | RMSD (Å) | Role in antimalaria activity | References | ||
---|---|---|---|---|---|---|
X | Y | Z | ||||
Lactate dehydrogenase (1ldg) | 25.329 | 23.422 | 41.843 | 1.2875 | Relating to the anaerobic nature of parasite life | Dunn et al. (1996) |
M1 Alanylaminopeptidase (3ebh) | 14.248 | 11.055 | 12.141 | 1.4307 | Involved in the terminal stages of hemoglobin digestion and essential for the provision of amino acids used for parasite growth and development within the erythrocyte. | McGowan et al. (2009) |
Plasmepsin II (5yib) | 18.733 | −25.663 | −24.032 | 1.9086 | Inhibition plasmepsin protein correlated with hemoglobin catabolism in which parasite survival was depended on host haemoglobin consumption | Mishra et al. (2018) |
PfNDH2 (5jwc) | 29.304 | 10.210 | 25.414 | 1.4617 | Inhibition of PfNDH2 will inhibit the reaction of ubiquinol formation, which will interfere with the parasite’s respiratory system | Yang et al. (2017) |
Falcylisin (8ho4) | −32.065 | 5.463 | −11.464 | 0.7339 | Inhibition of falcylisin disrupt the short polypeptide cleavage process, resulted accumulation of short poplypeptide in organel | Lin et al. (2024); Wirjanata et al. (2024) |
3. RESULT and DISCUSSION
The extract yield significantly differed for different woody stem parts of S. ligustrina (p < 0.05). The heartwood exhibited the highest extract yield, followed by the bark and sapwood (Fig. 1). A higher yield value of heartwood than sapwood was also reported in previous research using Quercus faginea (Miranda et al., 2017). The difference in yield is attributable to the different types and phytochemical compositions (Morais and Pereira, 2012). Therefore, if the heartwood and sapwood are not separated, then the extract yield will be diminished, particularly for woody stems with greater sapwood content. The considerable differences in extract yields among heartwood, sapwood, and bark provide valuable insights for optimizing extraction processes and maximizing the efficiency of wood resource utilization.
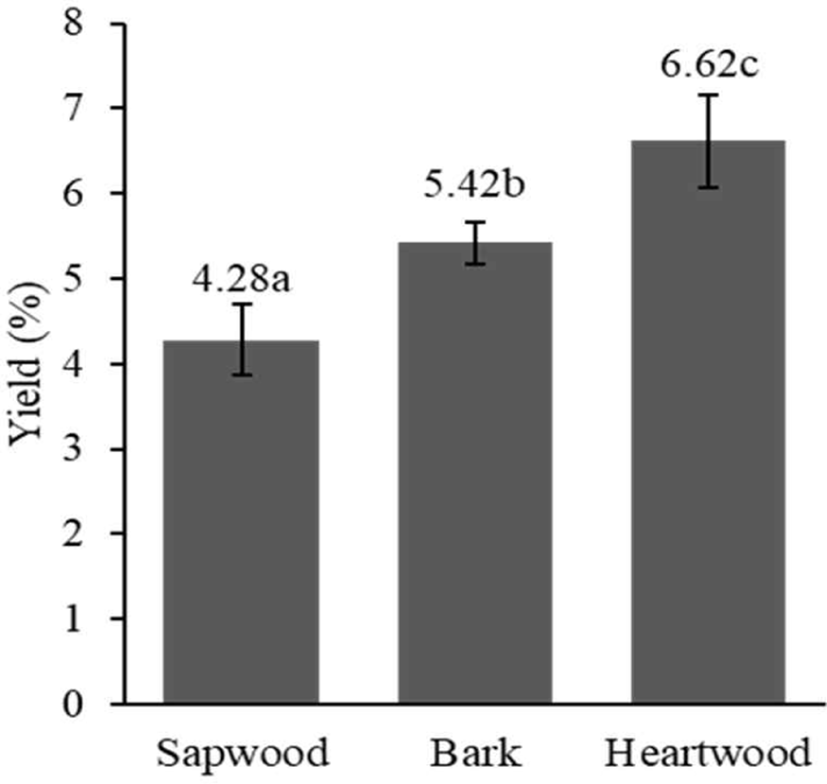
With the same extraction method, the yield of the aqueous extract of S. ligustrina wood in this study (4.28%–6.62%) was higher than that reported by Manurung et al. (2019; 3.62%). Differences in the wood diameter could cause a difference in yield. This research used S. ligustrina wood with a 14.5–17-cm diameter, whereas Manurung et al. (2019) used wood with a 7–10-cm diameter. The trees used in this study were older. The heartwood/sapwood ratio increases with increasing tree age, and the phytochemical content of heartwood from older trees is higher than that of younger trees (Arisandi et al., 2024; Canal et al., 2020).
The various woody stem parts of S. ligustrina influenced the phytochemical composition. According to the LC-MS/MS analysis, the chromatograms of heartwood, sapwood, and bark extracts were nearly identical, although the peak heights varied (Fig. 2). The identical chromatograms demonstrated that the phytochemical compounds in each extract were comparable, but their concentrations differed. This similarity could be observed at the retention time of 4–10 min. This range was considered a fingerprint area for the S. ligustrina wood extracts. Fig. 2 depicts the LCMS chromatograms, which consisted of 12 (heartwood), 13 (bark), and 10 peaks (sapwood). The most prominent spectral peaks, reflecting a molecular weight of 394.19 g/mol, were observed at retention times of 7.33 (heartwood), 7.14 (bark), and 7.53 min (sapwood).
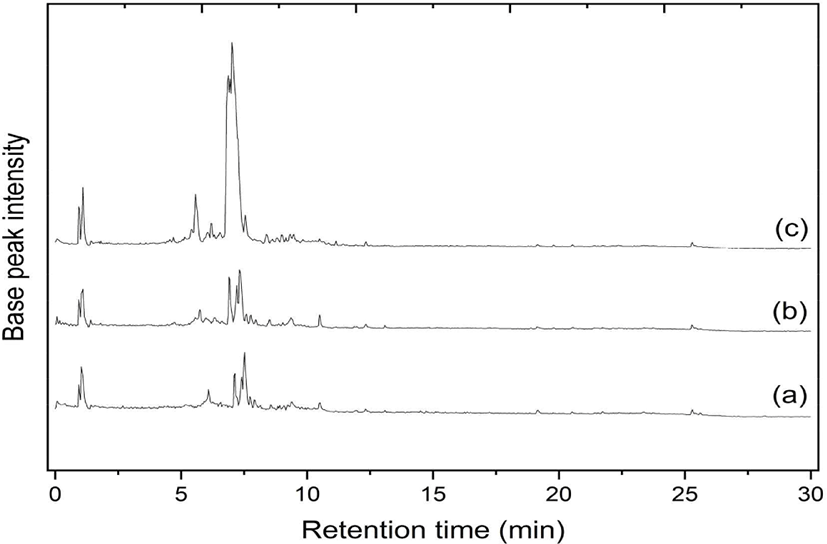
Sixteen compounds with a database similarity index greater than 70% were identified in the heartwood, sapwood, and bark extracts (Table 2). Alkaloid compounds were abundant in the three extracts. Heartwood and sapwood extracts had a similar compound composition, containing three highly abundant nitrogen-containing compounds (strychnine, choline, and brucine), with strychnine being predominant (Table 2). The compound composition of bark extract was dramatically different from those of the heartwood and sapwood extracts, with the most abundant compounds being brucine and N-[1-(6,7-dimethoxy-1,2,3,4-tetrahydroisoquinoline-1-ylidene)-4-methyl-2-oxopentan-3-yl]benzamide. Syafii et al. (2016) reported that the alkaloids brucine and strychnine were present in the wood ethanol extract of S. ligustrina and that they exhibit potent antimalarial activity. Brucine was also detected in other Strychnos species. Khasanah et al. (2024) described the presence of brucine in an S. lucida extract, and its content was correlated with antimalarial activity using an animal model. However, no other report detected N-[1-(6,7-dimethoxy-1,2,3,4-tetrahydroisoquinoline-1-ylidene)-4-methyl-2-oxopentan-3-yl]benzamide, which displayed high relative abundance in the bark of S. ligustrina.
The in vitro antimalarial assay demonstrates that all extracts could inhibit the growth of P. falciparum strain 3D7 (Fig. 3). Fig. 3(a–c) highlighted the concentration-dependent inhibitory effects of the extracts. However, the strength of the inhibitory effects of the bark, sapwood, and heartwood extracts varied by component. As presented in Fig. 3, 50% parasite growth inhibition was not achievable at extract concentrations of 0.01–10 μg/ mL. Meanwhile, growth was restricted by more than 50% by concentrations of 50 and 100 μg/mL, with 100 μg/mL exhibiting the highest inhibition.
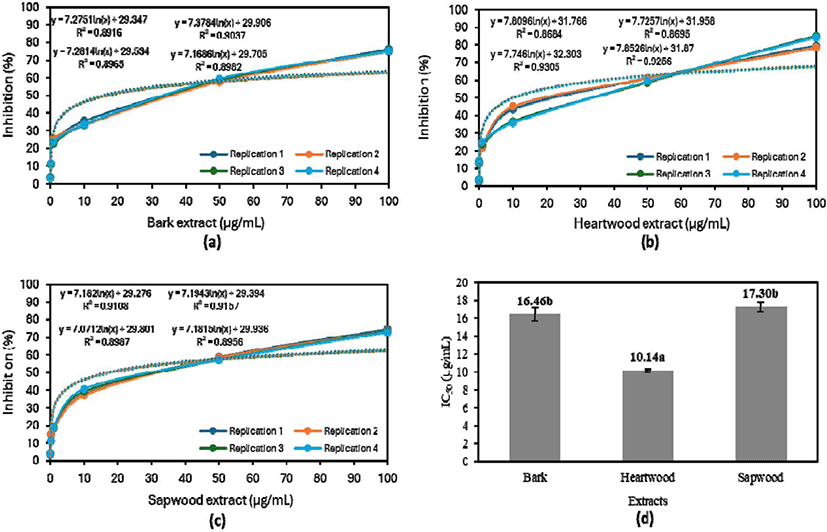
The antimalarial activity of aqueous extracts of various parts of S. ligustrina varied, with the IC50 ranging from 10.14 to 17.30 μg/mL. Fig. 3(d) illustrates that the aqueous heartwood extract, with an IC50 of 10.14 μg/mL, had the strongest antimalarial activity, followed by the bark and sapwood extracts. The IC50 values of the sapwood and bark extracts were not significantly different, although the value was slightly lower for the wood extract. This distinction is attributable to the nature and composition of the extractive components present in each extract (Miranda et al., 2017). Alkaloids might be responsible for the extracts’ effectiveness against malaria (Bekoe et al., 2022; Belete et al., 2020; Manurung et al., 2019; Syafii et al., 2016).
The phytoconstituents of S. ligustrina extracts exhibit low to high binding affinity for five proteins used from P. falciparum. The phytochemical compounds derived from the three extracts have varying binding affinity values towards the five target protein. Table 3 illustrates that Brucine showed the best potential for lactate dehydrogenase, while DCA had the strongest interaction with M1 alanylaminopeptidase and plasmepsin II. Meanwhile, the PfNDH2 and falcilysin proteins showed the highest binding affinity with 3,4-bis(4-propan-2-ylanilino)cyclobut-3-ene-1,2 dione and strychnine, respectively. These compounds exhibited a higher binding affinity than chloroquine, a commonly used antimalarial drug (Tse et al., 2019). To date, no studies have confirmed the in vitro mechanisms of these compounds binding to the five proteins used in this study. However, the potential of alkaloid compounds as antimalarials has been widely studied, yielding promising results (Uzor, 2020). These three compounds, which have different molecular structures, are classified as alkaloids. This structural difference results in varying binding affinities, which are also related to their interactions with the target proteins.
An analysis of the interactions of the compounds with the best binding affinities with receptors revealed differences in the interactions formed by the best-docked compounds compared to chloroquine as a positive control. In lactate dehydrogenase, brucine formed hydrogen bonds with Val248 and Arg171, while chloroquine did not form any hydrogen bonds [Fig. 4(a)]. A similar phenomenon was observed in falcilysin, where strychnine formed hydrogen bonds with Tyr413, but no hydrogen bonds were observed in the chloroquine-falcilysin interaction [Fig. 4(c)]. A different pattern was noted in the chloroquine-PfNDH2 interaction, where hydrogen bonds were present but fewer than in the BCD-PfNDH2 interaction [Fig. 4(b)]. As the active ingredient in antimalarial medications, chloroquine binds selectively to P. falciparum lactate dehydrogenase via NADH binding (Penna-Coutinho et al., 2011). Wirjanata et al. (2024) also reported that chloroquine binds to and inhibits the function of falcilysin. However, this study showed that many other phytochemical compounds have greater potency than chloroquine.
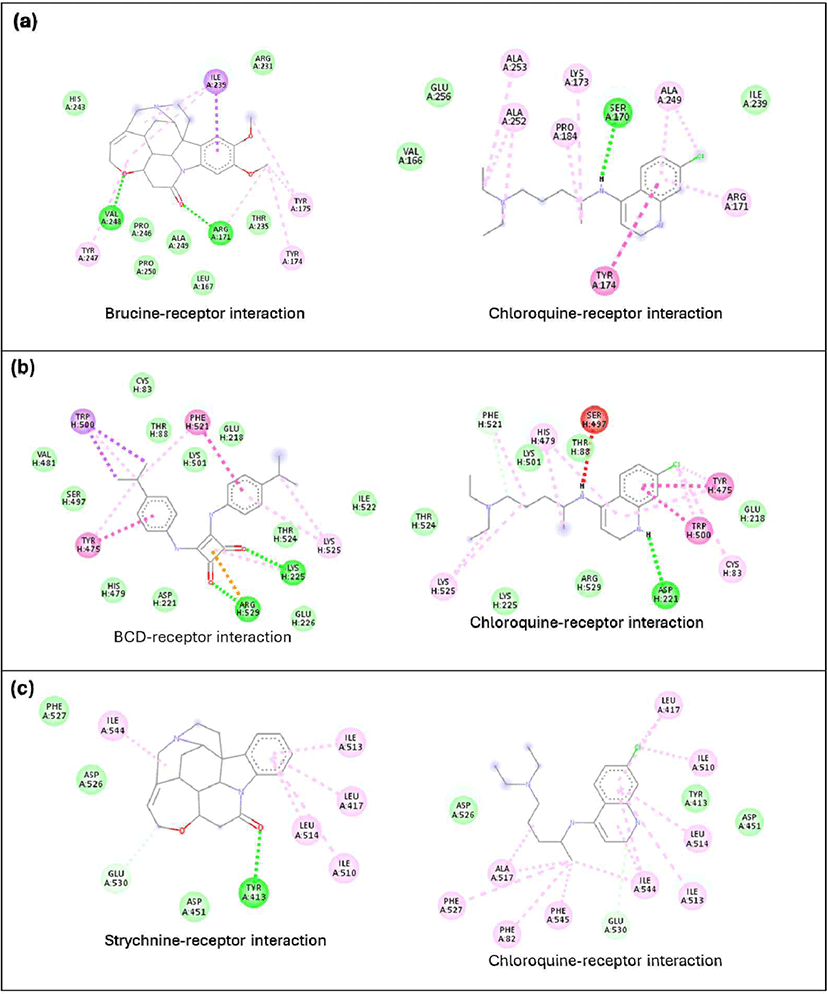
The DCA compound, which is the best-docked compound for M1 alanylaminopeptidase and plasmepsin II, showed differences in the interactions formed with each protein. The DCA interaction with M1 alanylaminopeptidase formed more hydrogen bonds [Fig. 5(a)]. Additionally, an important interaction in the DCA-M1 alanylaminopeptidase complex was the formation of a salt bridge. This interaction is believed to be a key factor in stabilizing the DCA-M1 alanylaminopeptidase complex. The stabilizing effect of salt bridges has also been confirmed through molecular dynamics studies on N-myristoyltransferase inhibitors, related to the treatment of protozoan infections (Spassov et al., 2023). However, no studies have discussed the formation of salt bridges by DCA with M1 alanylaminopeptidase. The interaction with plasmepsin II protein showed a similar number of hydrogen bonds formed but with different amino acid residues. DCA formed more pi-pi interactions than chloroquine, which is believed to contribute to better complex formation.
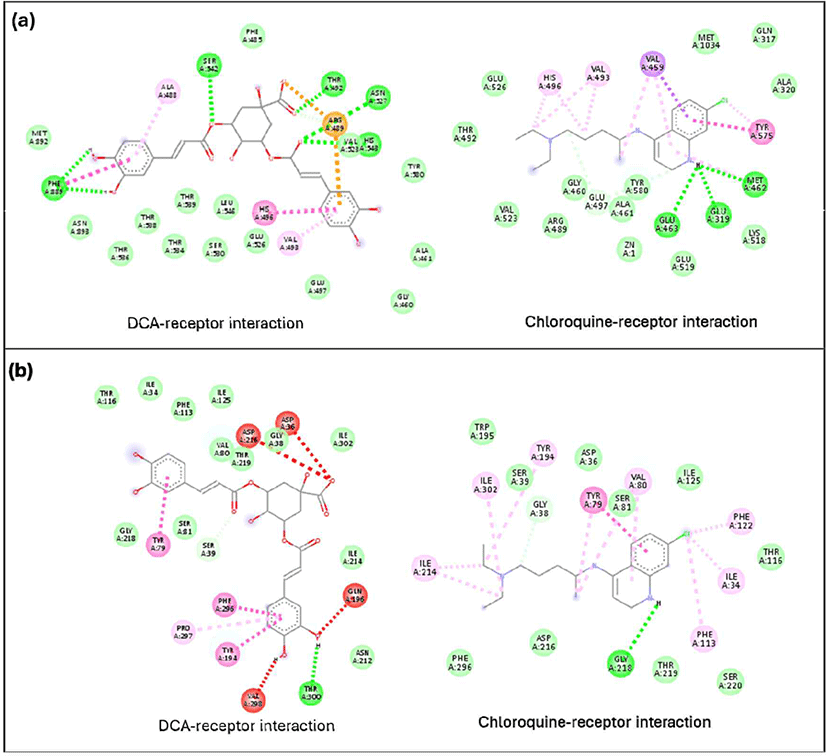
The high strychnine content in heartwood is presumed to be the main factor contributing to the best antimalarial activity of heartwood extract. The heartwood extract contains more strychnine than the sapwood and bark extracts (Table 2). This compound also showed the highest binding affinity with falcilysin protein. Additionally, strychnine exhibited relatively high binding affinity with M1 alanylaminopeptidase and lactate dehydrogenase (Table 3). Based on this, the targeting of falcilysin protein is predicted to be the primary mechanism behind the antimalarial properties of heartwood extract. Philippe et al. (2006) reported the potent antiplasmodial activity of strychnine targeting the glycine receptor, which is highly correlated with the ether bond in the G-cycle of the strychnine molecular structure.
All the extracts studied in this research had IC50 values below 20 μg/mL [Fig. 3(d)], indicating that they are all effective as antimalarials. Therefore, it is believed that these extracts may have specific mechanisms of action due to the distinct composition of compounds found in the sapwood and bark extracts. The sapwood has a compound profile similar to the heartwood, suggesting that its mechanism of action is likely similar to that of the heartwood extract (Table 2). In contrast, the bark extract has a composition significantly different from both the sapwood and heartwood. The bark contains high concentrations of brucine and BCD (Table 2), which showed the best binding affinity to lactate dehydrogenase and PfNDH2, respectively (Table 3). The NTB compound also has a high abundance in the bark extract (Table 2), and this compound has a fairly high binding affinity for plasmepsin and PfNDH2 proteins (Table 3). This suggests that the antimalarial activity of the bark extract is related to the inhibition of several proteins. Additionally, the compound DCA, identified as the best-docked compound in M1 alanylaminopeptidase [Fig. 5(a)], was present at low concentrations in all three extracts, indicating that it may also contribute to the antimalarial activity across the extracts.
Although some compounds may have stronger or weaker activities, each compound contributes to antimalarial activity because the study used an extract containing various of compounds. Study Na and Kim (2022) and Yoon and Kim (2023) proved that a combination of extracts with a greater variety of compounds than a single extract had higher antifungal activity than a single extract. Some compounds have been shown to work synergistically as antifungals. However, there is a tendency for certain proteins to have a more significant effect than other proteins. It is confirmed through the heartwood extract, which has the highest levels of strychnine (Table 2) and the highest binding affinity to falcylisin (Table 3). It is believed to enhance its antimalarial effects. In addition, the high content of brucine in the bark also had a good effect on the antimalarial activity of the bark extract (IC50 < 20 μg/mL). However, the effect was not as good as that of strychnine observed in the heartwood extract.
4. CONCLUSIONS
The extractive yields of S. ligustrina using a water-based solvent differed among the various parts of the wood, with the highest yield obtained from the heartwood extract. In terms of effectiveness, the heartwood extract demonstrated a stronger inhibition of P. falciparum compared to the sapwood and bark extracts. However, the IC50 values of all S. ligustrina extracts from different parts of the stem were below 20 μg/mL, confirming their potency as antimalarial agents. LC-MS/MS analysis indicated that, although the extracts shared a similar chemical composition, the concentrations of individual components varied. Strychnine was the dominant compound in both the heartwood and sapwood, while brucine was more prevalent in the bark. Strychnine, which is the most abundant compound in the heartwood, exhibited the strongest potential for binding to the falcilysin protein. Additionally, other compounds with strong molecular binding activity suggest potential for further exploration of these specific protein interactions.