1. INTRODUCTION
The Lauraceae family consists of aromatic trees that are predominantly found in Asia as well as North and South America. There are approximately 2,500–3,500 species distributed throughout the world (Silva et al., 2009). Many species of the Lauraceae family have commercial uses, such as in the food, pharmaceutical, and perfume industries (Chau et al., 2020). Previous studies reported biological activities, such as antioxidant, antifungal, antibacterial, anti-inflammatory, anti-tyrosinase, antileishmanial, anticholinesterase, anti-melanogenic activity, etc., for the essential oils (EOs) isolated from the Lauraceae family (Damasceno et al., 2019).
Natural products are increasingly popular with the growing interest in human health (Min et al., 2017). The EO is one of the secondary metabolites derived from aromatic plants, and it consist of volatile compounds (Ham et al., 2020). The EOs can relieve certain symptoms including anxiety, pain, insomnia, and bacterial infections (Ali et al., 2015; Kim et al., 2020). It is known to have bioactivities such as anti-fungal, anti-insect, anti-bacterial, and anti-inflammatory, and antioxidant activities (Jang et al., 2012; Lee et al., 2014; Pujiarti and Kasmudjo, 2016; Yang et al., 2019; Yang et al., 2022). EOs obtained from some species have been traditionally used to treat various respiratory ailments, which are associated with mucus hypersecretion and accumulation in the airways, such as asthma and chronic obstructive pulmonary disease (Asif et al., 2020; Thai et al., 2008). However, there are no reports demonstrating an inhibitory effect of the EO of the Lauraceae family on mucus secretion in the airway.
Asthma is a common chronic disease that affected an estimated 334 million people worldwide, it causes approximately 25 million deaths annually (Bonser and Erle, 2017). Asthma causes symptoms such as wheezing, coughing, and chest tightness due to airflow obstruction caused by inflamed airway smooth muscle and impaired mucosal (Fanta, 2009). Airway mucus hypersecretion is a feature of many asthmatics and indicates poor asthma control (Rogers, 2004). Excessive mucus production not only obstructs airways but also contributes to airway hyperresponsiveness (Evans et al., 2009). Therefore, various studies on anti-hypersecretory agents for regulating airway mucus hypersecretion in asthma are currently being conducted (Ha and Rogers, 2016).
Inflammatory mediators [interleukin (IL)-1β and IL-6] commonly upregulated during respiratory inflammatory responses increase MUC5AC mRNA in lung epithelial cells and transcriptionally upregulate MUC5AC gene expression (Chen et al., 2014). This interaction suggested that pro-inflammatory cytokines could induce mucin gene expression by cytokine receptors with the regulatory regions of the mucin gene (Wang et al., 2007). Mucins are high-molecular weight glycoproteins and are the predominant constituents that impart the viscoelastic and gel-forming properties to mucus (Williams et al., 2006). The 20 mucin genes that are known to be expressed in the lungs include MUC1, -2, -4, -5AC, -5B as well as others (Rose and Voynow, 2006). Of these, MUC5AC and MUC5B are considered the predominant mucins in airway mucus, because they are the only ones consistently found to be expressed at the protein level (Thornton et al., 2000). This study was determined whether EOs can suppress mucin gene expression in NCI-H292 epithelial cells in vitro.
In this study, the EOs from seven species [Cinnamomum camphora (L.) J. Presl, Cinnamomum cassia (L.) J. Presl, Cinnamomum japonicum Siebold, Lindera obtusiloba Blume, Machilus japonica Siebold & Zucc., Neolitsea aciculata (Blume) Koidz., and Neolitsea sericea (Blume) Koidz.] in Lauraceae were evaluated the inhibitory effects on the pro-inflammatory cytokines and mucin genes. In addition, the chemical composition of EOs were investigated using gas chromatography-mass spectrometry.
2. MATERIALS and METHODS
The leaves of C. camphora (L.) J. Presl, C. cassia (L.) J. Presl, C. japonicum Siebold, N. aciculata (Blume) Koidz., and N. sericea (Blume) Koidz. were collected from Jeju Island. The leaves of L. obtusiloba Blume and M. japonica Siebold & Zucc. were obtained from Yeongju and Wando, respectively. Information on collection sites is summarized in Table 1.
The Lauraceae family EOs were extracted from the leaves of C. camphora, C. cassia, C. japonicum, L. obtusiloba, M. japonica, N. aciculata, and N. sericea by hydro-distillation method. A 10 L round-bottom flask containing 1.0 kg based fresh weight of the leaves was poured 6 L of distilled water and placed on a digital heating mantle (MS-DM608 heating mantle, MTOPS®, Misung Scientific, Yangju, Korea), then connected to the Clevenger-type apparatus. To prevent the volatile components of the EOs from evaporating, a refrigerant was circulated in the condenser to –5°C and the mixtures were heated to 104 ± 2°C for 6 h. The EOs were stored at 4°C until used. The EO yield was determined by the following Equation (1).
The volatile components of the EOs were analyzed using Trace 1300/ISQ-LT gas chromatography-mass spectrometry (GC-MS, Thermo Fisher Scientific, Waltham, MA, USA) fitted with a Tr-5MS column (30 m × 0.25 mm × 0.25 μm; Thermo Fisher Scientific). The injector temperature was set at 250°C, and helium (He, 1.0 mL/ in, 25 psi) was used as the carrier gas. The oven temperature was programmed to 40°C for 3 min and then increased to 200°C at a temperature increase rate of 3°C/min. The temperature was then raised at 15°C/min to 340°C and maintained for 10 min. Flame ionization detector (FID) signals and mass spectra of the detected compounds were recorded. The FID was set at 280°C and the flow rate of the helium make-up gas was 40.0 mL/min. The temperature of the mass spectrometer interface and the ion source were 280°C and 250°C, respectively. The mass spectra were recorded at a rate of 0.2 scans/s in the EI-scan range of 35–360 m/z. n-alkanes (C7–C29, Sigma-Aldrich, St. Louis, MO, USA) were used to calculate the retention indices of the components. Compared with the NIST 11 (National Institute of Standards and Technology, Gaithersburg, MD, USA) mass spectral library, the compounds with the highest match values were listed as the compound names.
The human bronchial epithelial cell line NCI-H292 (KCLB No. 21848) was obtained from the Korea Cell Line Bank (Seoul, Korea). NCI-H292 cells were cultured in RPMI-1640 (Welgene, Gyeongsan, Korea) supplemented with 10% fetal bovine serum (FBS, Gibco, Grand Island, NY, USA), 1% penicillin-streptomycin (Gibco), and 0.4 μL/mL plasmocin (InvivoGen, San Diego, CA, USA) at 37°C in a 5% CO2 incubator (Panasonic, Osaka, Japan).
Cell cytotoxicity of seven EOs on NCI-H292 cells was examined using Cell Counting Kit-8 (CCK-8, DoGenBio, Seoul, Korea) according to the previous study (Yang et al., 2021a). The EOs were prepared in dimethyl sulfoxide (DMSO, Sigma-Aldrich). The cells were seeded into 96-well plates at a density of 8,000 cells/well and incubated at 37°C in a 5% CO2 incubator for 24 h. Thereafter, the culture medium was removed and washed with Dulbecco’s phosphate-buffered saline (DPBS, Gibco). The cells were treated with concentration at 10–7–10–4% of the EOs and cultured under the same conditions described above. After treatment, the cells were washed twice with DPBS and treated with 100 μL of 10% CCK-8 reagent. The plates were then incubated at 37°C for 1 h. Then, the optical density value of the plates was measured using a microplate reader (Epoch, BioTek Instruments, Winooski, VT, USA) at 450 nm. The cell viability was calculated using the following Equation (2).
Where, At is the absorbance of treated cells, Ab is the absorbance of the blank, and Ac is the absorbance of the control.
Total RNA was isolated from NCI-H292 cells as done in a previous study (Yang et al., 2021b). NCI-H292 cells were cultured in 6-well plates (1 × 106 cells/well) for 24 h. After washing the cells with DPBS, the cells were stimulated with 1 μg/mL lipopolysaccharide (LPS, Sigma-Aldrich) for 1 h and then washed twice with DPBS. The cells were treated with 5 μg/mL budesonide and the seven EOs diluted at an appropriate concentration for 24 h. The cells were washed twice with DPBS and RNA was isolated using TRIzol reagent (Invitrogen, Waltham, MA, USA) and quantified using a Nanodrop 2000 spectrophotometer (Thermo Fisher Scientific) at 260 nm. Each cDNA samples were synthesized from the RNA and quantitative analysis was carried out using a CFX96 real-time PCR (Bio-Rad Laboratories, Hercules, CA, USA). The reaction volume was 10 μL containing 5 μL SYBR® reaction mix (1X final, Bio-Rad Laboratories), 1 μL each primer (3 pmol), 1 μL DNA template, and 2 μL distilled water. Thermal cycling was performed with an initial denaturation at 95°C for 1 min followed by 40 cycles of denaturation at 95°C for 10 s, and an annealing step at 60°C for 30 s. The oligonucleotide primers used for PCR amplification are listed in Table 2.
Each experiment was performed at least three times. Statistical analysis was done using a repeated one-way analysis of variance with Statistical Package for the Social Sciences software (SPSS ver. 17.0, SPSS, Chicago, IL, USA). Values are expressed as the mean ± SD. p values less than 0.05 were considered statistically significant.
3. RESULTS and DISCUSSION
EOs from seven species belonging to the Lauraceae family were obtained by hydro-distillation. The yields were 1.45 ± 0.64% in C. camphora leaves, 0.58 ± 0.0% C. cassia leaves, 0.57 ± 0.06% in C. japonicum leaves, 0.25 ± 0.05% in L. obtusiloba leaves, 0.33 ± 0.06% in M. japonica leaves, 0.28 ± 0.09% in N. aciculata leaves, and 0.38 ± 0.16% N. sericea leaves. The highest yield was observed in C. camphora and the lowest in L. obtusiloba.
The chemical composition of the EOs is summarized in Table 3 only for components with 3% or more. The chemical composition of EOs depends on the plant species, even if they belong to the same family (Ahn et al., 2018). The major component of EO from C. camphora was camphor (89.09%). Linalool (26.91%), (E)-citral (10.69%), and (Z)-citral (8.46%) were identified as the major components of EO from C. cassia. The major components of EO from C. japonicum were 1,8-cineole (23.90%), α-phellandrene (11.75%), and β-myrcene (9.04%). D-limonene (10.27%) and β-eudesmol (10.03%) were major components in EO from L. obtusiloba. The major components of EO from M. japonica were δ-cadinene (13.85%), α-phellandrene (11.57%), and τ-muurolol (9.52%). cis-, trans-β-Ocimene (13.80% and 12.06%), elemol (11.46%), and β-elemene (10.94%) were identified as the major components of N. aciculate oil. The major component of EO from N. sericea were cis-β-ocimene (37.94%) and sabinene (24.91%). In case of C. camphora oil, M. japonica oil, and N. sericea oil, the results in the present study were similar to the results of previous studies (Pragadheesh et al., 2013; Van Khien et al., 2009; Yoon et al., 2010). However, the results of C. cassia oil, C. japonicum oil, L. obtusiloba oil, and N. aciculata oil were different from the result of previous study (Chahbi et al., 2020; Hwang et al., 2013; Kim et al., 2011; Zhang et al., 2018). The major components of C. cassia oil, C. japonicum oil, L. obtusiloba oil, and N. aciculata oil in previous studies were found as minor component in this study. There was a difference in chemical composition of EOs according to the growing environment of plants (Chrysargyris et al., 2022). Especially, chemical compositions are related to exogenous factors such as light, geographical location, precipitation, harvesting time, and soil conditions (Şanli and Karadoğan, 2017; Zouari et al., 2012). When plants are exposed to other exogenous factors for a long time, it could undergo genetic modification, which causes a change in biosynthetic pathways, leading to the qualitative/quantitative variation of secondary metabolites (Barra, 2009).
Natural products can interact with DNA and cause changes in its structure of function, so the appropriate concentration of EO should be determined by assessing the cell cytotoxicity (Ahn et al., 2021). To determine the cell toxicity of the EOs, NCI-H292 cell viability was measured at various concentrations (10–7%–10–4%) of the seven EOs (Fig. 1). The cell viability was more than 80% at concentrations of 10–7%–10–4%, and no cytotoxicity was observed at concentrations lower than 10–4%. Based on the cytotoxicity results, the inhibitory effect on gene expression was performed at a concentration of 10−7%.
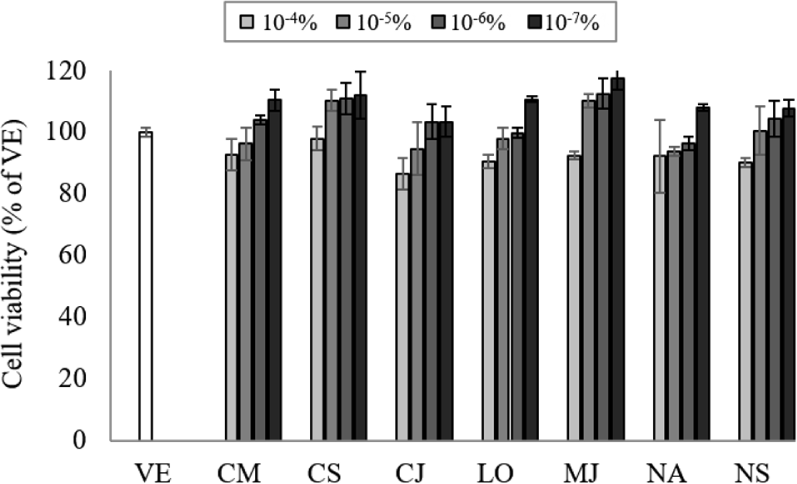
Several cytokines induce MUC5AC and MUC5B gene expression and mucus hypersecretion (Kim, 2007). The inflammatory mediators such as IL-1β and IL-6 are responsible for mucin hypersecretion in inflammatory diseases of the lung and gastrointestinal tract (Van Seuningen et al., 2001). The EOs from the seven species significantly inhibited the expression of the pro-inflammatory cytokines in LPS-stimulated NCI-H292 cells (Fig. 2).
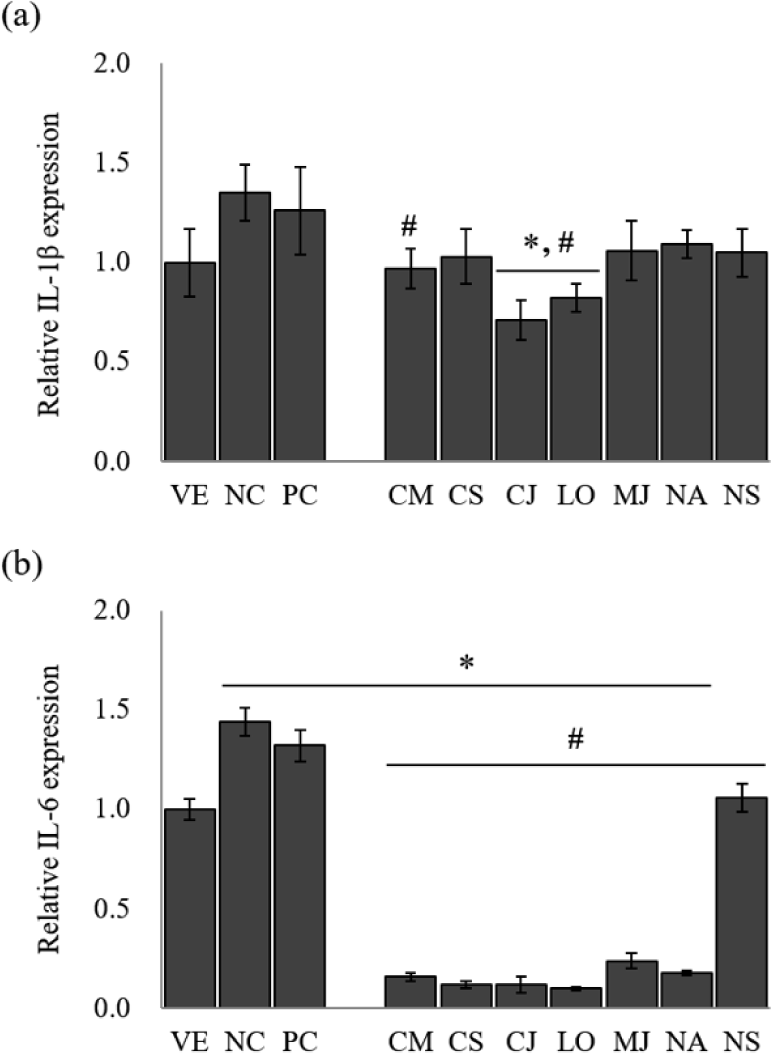
The IL-1β gene expression of LPS-stimulated NCI-H292 cells [negative control (NC)] was approximately 1.4-fold higher than that of the untreated cells [vehicle (VE); Fig. 2(a)]. A positive control (PC) group treated with budesonide down-regulated gene expression by 6.7% compared to NC. The treatment of EOs on LPS-stimulated NCI-H292 cells reduced IL-1β gene expression by 19.3%–47.4% compared to NC. Among seven species, IL-1β gene expression showed the highest inhibitory effect in C. japonicum and L. obtusiloba, with 47.4% and 39.3% reduction, respectively.
LPS-stimulated NCI-H292 cells significantly increased the IL-6 gene expression level [Fig. 2(b)]. However, treatment with budesonide suppressed the IL-6 gene expression compared with NC by 8.3%. Similarly, treatment with EOs significantly reduced the IL-6 gene expression by 26.4%–93.1%. The EOs of L. obtusiloba (about 93.1% reduction), C. japonicum (about 91.7% reduction), and C. cassia (about 91.7% reduction) effectively inhibited the IL-6 gene expression from the LPS-stimulated NCI-H292 cells.
IL-1β is a prototypical inflammatory cytokine implicated in many inflammatory diseases and is a useful probe to evaluate mucin secretion. In particular, it is known to stimulate mucin gene MUC5AC expression (Gray et al., 2004). IL-6 has also been reported to induce MUC5AC gene expression (Hashimoto et al., 2004). These results suggest that EOs of L. obtusiloba and C. japonicum have a possibility for the suppression of hypersecretion of airway mucus in asthma. However, further studies will be performed to clarify the effect of each component of EOs on the asthma animal model.
MUC5AC and MUC5B, the predominant gel-forming mucins, have been informed to be expressed in human airway epithelium, and are known as the predominant gel-forming mucins in normal or pathologic airway secretions (Henke et al., 2003; Okuda et al., 2019). To identify the inhibitory effect of mucus hypersecretion, the expression of the MUC5AC and MUC5B genes was evaluated in response to treatment with the seven EOs (Fig. 3).
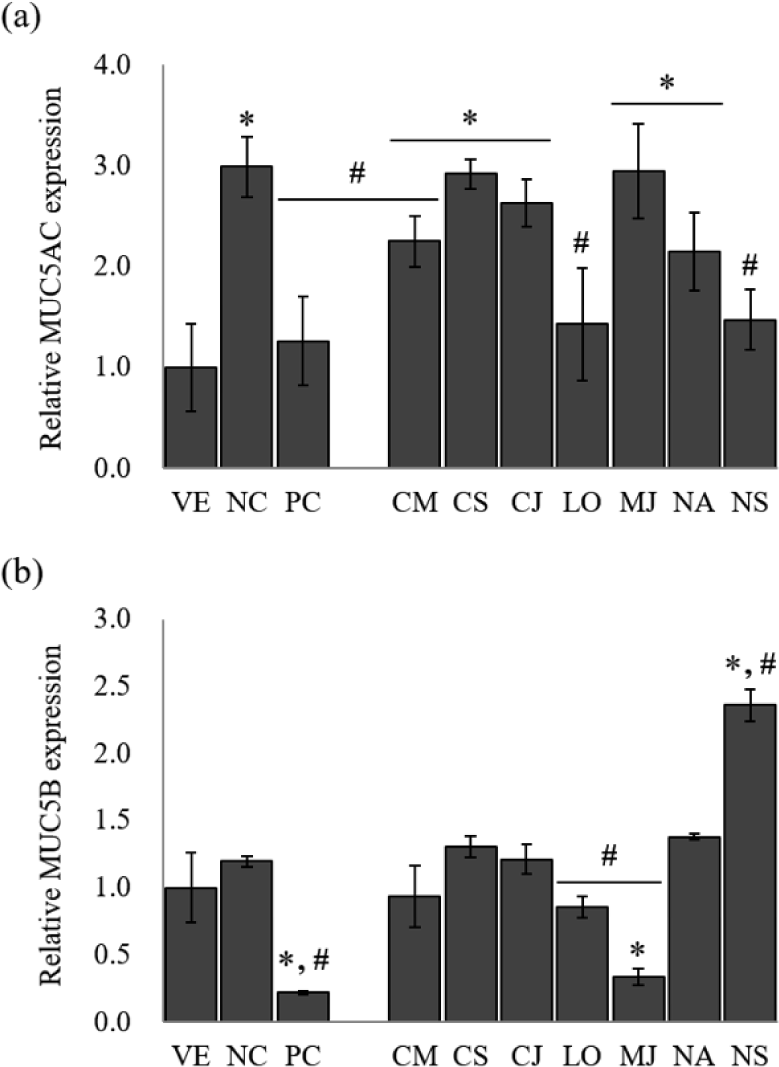
The NC group showed a remarkable increase of 3.0-fold in MUC5AC gene expression compared to LPS-stimulated NCI-H292 cells. Budesonide inhibited the MUC5AC expression by 57.9% compared to NC. The treatment of EOs reduced MUC5AC gene expression by 1.3%–52.2% compared to NC. Among the EOs, MUC5AC gene expression showed the highest inhibitory effect by L. obtusiloba and N. sericea, with 52.2% and 50.8% reduction, respectively.
In the case of MUC5B, another secretory mucinous, the EO inhibited the gene expression of MUC5B, effectively. MUC5B expression was increased by 1.2-fold in the LPS-stimulated NCI-H292 cells compared to VE. Budesonide, the PC, reduced the relative expression of the mucin gene by 81.7%. Among the seven EOs, only M. japonica, L. obtusiloba, and C. camphora inhibited the MUC5B gene expression. The EOs of M. japonica showed a superior inhibitory effect of the MUC5B gene expression by 71.7% reduction than other EOs.
Among the seven EOs, L. obtusiloba significantly suppressed MUC5AC and MUC5B expression (p < 0.05). L. obtusiloba is distributed in the north and southeast regions of Asia and has been used in traditional Chinese, Korean, and Japanese medicine over centuries (Lim et al., 2016). In Korea and China, it is traditionally used for restoring blood stasis and inflammatory disorders (Kim et al., 2016). Also, the leaves of L. obtusiloba are traditionally consumed as both tea and food (Oak et al., 2014). In a previous study, a methanolic extract of L. obtusiloba inhibited airway inflammation in a murine model of ovalbumin (OVA)-challenged asthma, suggesting that it may have possible applications in allergic diseases, such as asthma and atopic dermatitis (Lee et al., 2020). Methanolic extract of L. obtusiloba consisted of quercetin rhamnoside (26.04 ± 0.05 mg/g) and kaempferol rhamnoside (15.82 ± 0.02 mg/g), and an unknown compound. L. obtusiloba extract treatment significantly suppressed the MUC5AC expression in the lung tissues compared with the OVA-challenged asthma model. When L. obtusiloba extract (50 or 100 mg/kg) was treated in the OVA-challenged asthma model, MUC5AC expression was decreased by 3.2% and 32.3%, respectively. Compared with the results of this study, it was shown that the mucin-inhibitory effect of L. obtusiloba EO was superior to that of the extract. However, the effects of L. obtusiloba oil and the active components have not been reported. Table 2 shows that D-limonene (10.27%), β-eudesmol (10.03%) and hedycaryol (6.79%) are the predominant components in the L. obtusiloba oil. Limonene was known to be effective in reducing allergic airway inflammation through previous studies (Hirota et al., 2012; Narke et al., 2018). The chemical composition was compared with other Lauraceae family EOs. The portion of limonene was the highest in L. obtusiloba EO. Therefore, it is considered that the superior anti-asthmatic activity of L. obtusiloba EO is derived from limonene.
4. CONCLUSIONS
Plant secondary metabolites represent a valuable resource that can be directly used for drug development or further modified into therapeutic agents. EOs from the Lauraceae family are known to have various bioactivities. This study evaluated the anti-asthmatic activity of EOs from seven species in the Lauraceae family. Among them, the EO from L. obtusiloba leaves significantly suppressed the expression of pro-inflammatory cytokines and mucin genes associated with allergic airway inflammation and mucous hyperplasia. These results are suggested that the EO of L. obtusiloba leaves can be used as an agent to suppress mucus hypersecretion. However, the active components that inhibit mucus hypersecretion remain to be identified. Further studies are necessary to explore the specific molecular targets of the active components of EOs in vivo.