1. INTRODUCTION
Lignin, one of the primary biomass components along with cellulose and hemicellulose, accounts 15 – 30 % by weight of the lignocellulosic biomass (Yang and Pan, 2016). In addition to the existing pulp and paper industries, a massive amount of lignin is expected to be collected from the emerging lignocellulosic biorefineries (Kim and Kim, 2018). From the technoeconomic standpoint, lignin valorization holds the key to success in the future biorefinery (Ragauskas et al., 2014). Despite the potential promise of lignin utilization, lignin is still poorly utilized due to its inherent recalcitrance.
There have been enormous efforts to overcome the technical barriers associated with lignin. In this regard, plant genetic engineering to alter lignin content and/or composition in the biomass cell wall structure has been widely studied (Mahon and Mansfield, 2019). As shown in Fig. 1, there are many enzymes participate in the phenylpropanoid pathway, many of them have been considerably examined. For example, studies reported that manipulating monolignol biosynthesis genes such as cinnamate 4-hydroxylase (C4H), cinnamoyl-CoA reductase (CCR), caffeic acid O-methyltransferase (COMT) and cinnamyl alcohol dehydrogenase (CAD) can produce biomass with structurally altered lignin. Strategic downregulation of CAD gene during the lignin monolignol biosynthesis pathway accumulates more p-coumaryl aldehyde, coniferyl aldehyde and sinapyl aldehyde. These three phenolic aldehyde precursors participate in lignin biosynthesis in the plant cell wall forming unique interunit linkages as shown in Fig. 2 (Zhao et al., 2013).
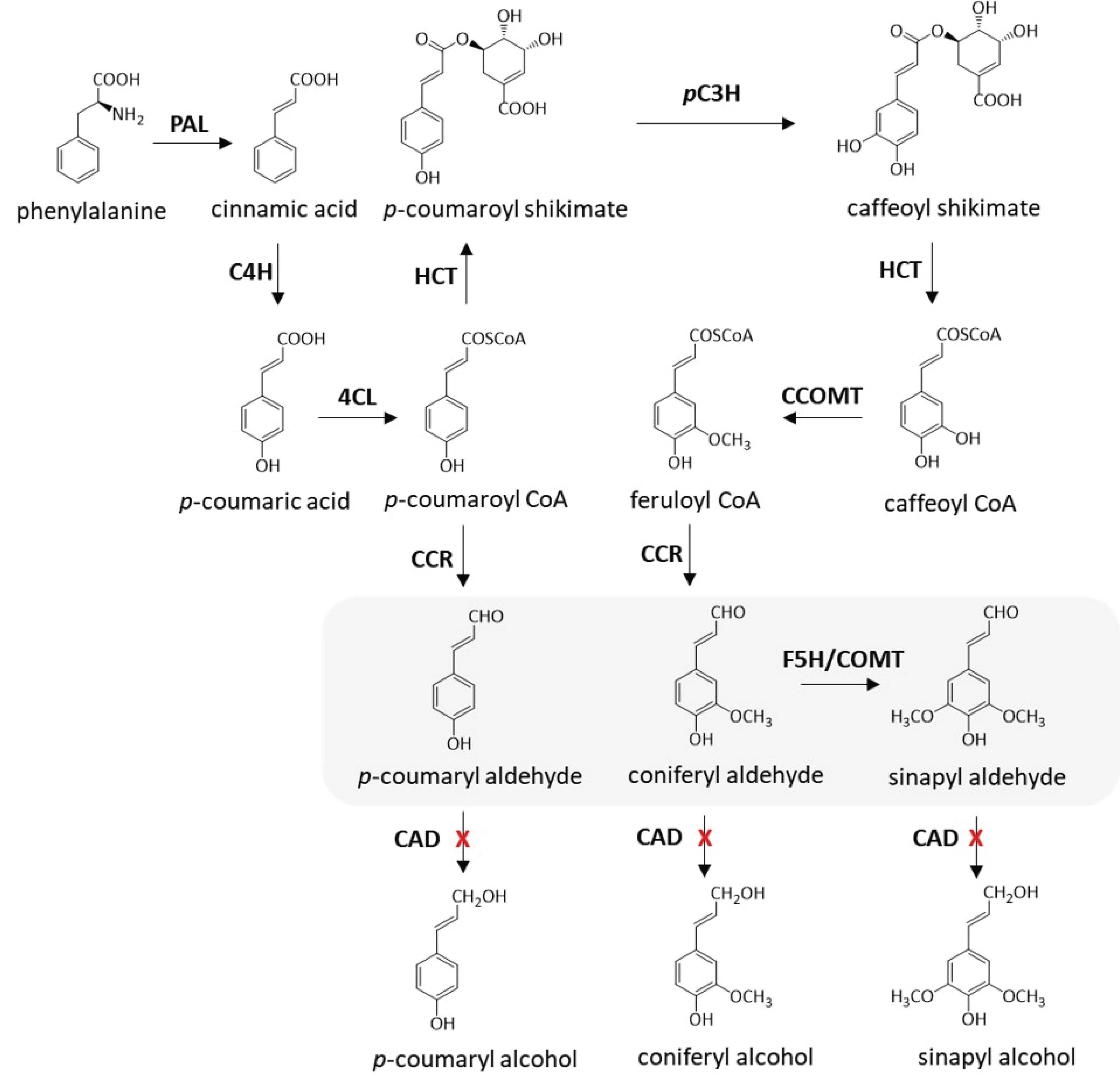
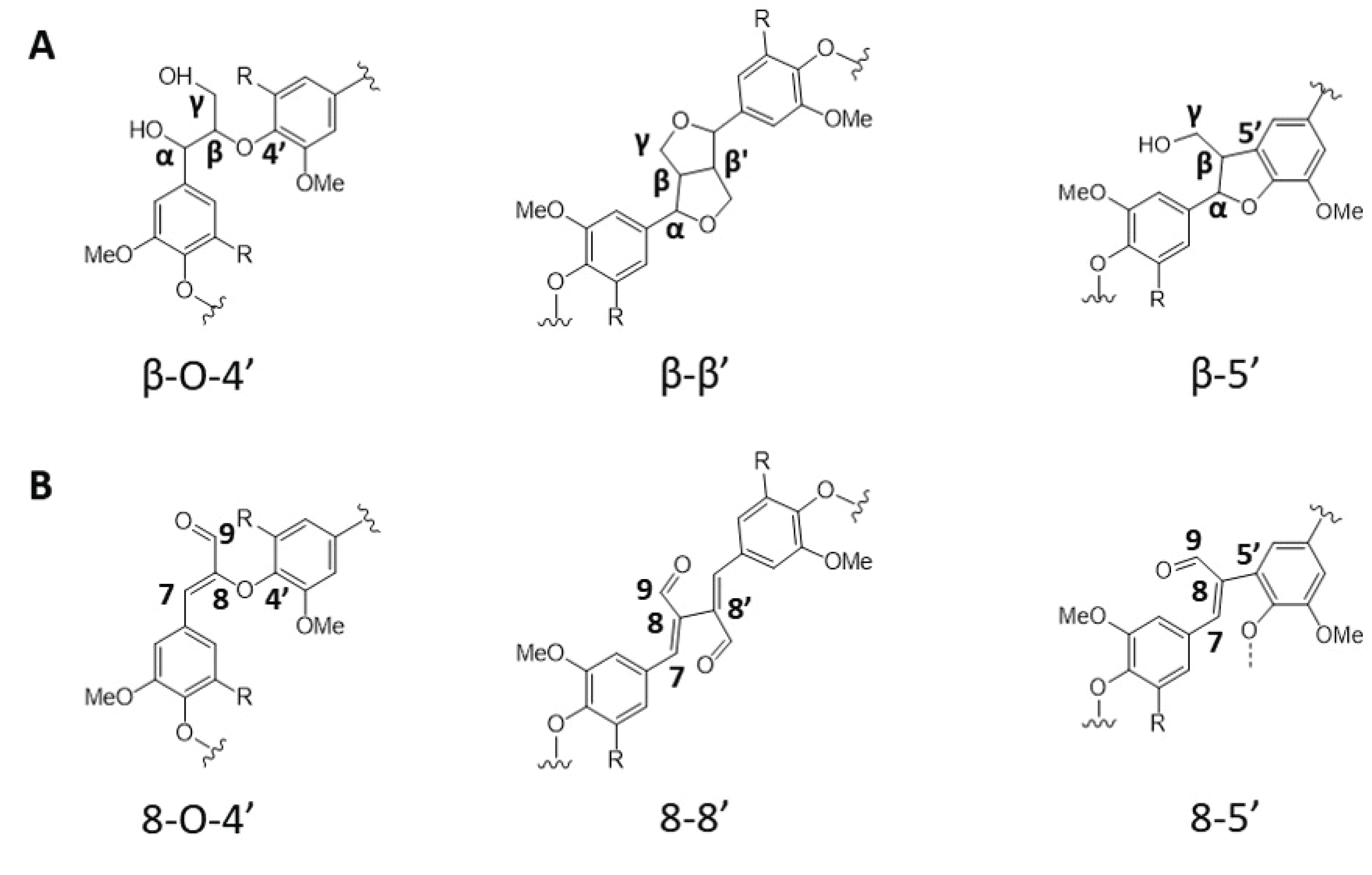
Fast pyrolysis of lignin has gained enormous attention as it can produce valuable phenol and phenolics (Kim, et al. 2011, Kim, et al., 2015a). However, reactions behind lignin pyrolysis are extremely complex and can be influenced by many factors including source, purity and interunit linkages of lignin. Although the pyrolysis of lignin has been studied over the past decades, only a few studies focused on the effect of genetic alteration of lignin on pyrolysis.
In this work, lignin samples extracted from WT and CAD downregulated biomass are pyrolyzed using an analytical pyrolyzer to understand how the strategic alteration of lignin composition affects the products yield and distributions. Also, we report the results of a computational study investigating the chemical reactivity of engineered lignin structure. This work could provide insights toward developing a biomass processing integrated with plant genetic engineering and thermochemical conversion technology.
2. MATERIALS and METHODS
Arabidopsis thaliana seeds from wildtype (WT) and CAD double mutant (cad-c and cad-d) were germinated directly on the soil. The details, including gene identification, plant growth and other analyses, have been previously described (Sibout et al., 2005). Lignin was extracted from both WT and CAD mutant biomass for pyrolysis test. Each biomass sample was finely ground and subjected to enzymatic hydrolysis, followed by mild acidolysis to extract pure lignin (Guerra et al., 2008). Physicochemical properties of both WT and CAD mutant lignin, including molecular weight and 2D HSQC NMR spectra for interunit linkage analysis, are well described in the literature (Kim et al., 2019).
A coil-type CDS Pyroprobe 5000 (CDS Analytical Inc., Oxford, PA, USA) was used in this study. In sample preparation, 0.7-0.8 mg of lignin was introduced to a quartz tube with 1.25 μL internal standard (IS; 3.9 mg of fluoranthene/mL of methanol) for quantification of pyrolysis products (Kim et al., 2013, Kim et al., 2015b). The sample was pyrolyzed at 400 and 500 °C with a heating rate of 10 °C/ms and maintained for 20 s at a final temperature in an inert atmosphere. During pyrolysis, the pyrolyzer interface and transfer line were maintained at 250 °C. The released pyrolysis products were on-line transferred to a GC-MS/FID instrument (Agilent Technologies 7890A/Agilent Technologies 5975A, USA) equipped with a DB-5 capillary column (30 m×0.25 mm ID×0.25 μm film thickness) with a split ratio of 1:100. The separated products were split equally to FID and MS system. The gas chromatograph injector and detector temperature were set at 250 °C and 300 °C, respectively. The oven temperature began at 50 °C for 5 min and then up to 280 °C with a heating rate of 3 °C/min. It maintained for 10 min at final temperature.
The MS source temperature and MS quad temperature was 230 °C and 150 °C, respectively. The separated products were introduced into the ionization source of a quadrupole MS. The ionization was achieved with electron impact (EI) mode at 70 eV. The mass spectrometer was scanned from m/z=50 to m/z = 550. Identification of each compound was based on the NIST MS Search 2.0 (NIST/EPA/NIH Mass Spectral Library; NIST 02) and proper reference (Faix et al., 1990). For quantitative analysis, the response factor (RF) between each authentic compound and the IS was determined by GC-FID information (Kim et al., 2015a).
Computational study was conducted to understand the chemical reactivity of WT and CAD mutant lignin. First, the geometry optimization of the typical β-O-4’ dimeric model compound found in WT (guaiacylglycerol-β -guaiacyl ether) and 8-O-4’ dimer in CAD mutant (guaiacylacrylaldehyde-β-guaiacyl ether) was carried out using density functional theory (DFT) with the B3LYP functional and the 6-31+G(d,p) basis set (Shi et al., 2016). Frequency calculation was conducted to verify that the optimized structures corresponded to energy minima. Herein, the DFT-based global electrophilicity index (ω, eV) was calculated using Gaussian 09 software (Socha et al., 2014). Equations for the calculation of the electronegativity (χ , eV) and the hardness (η, eV) is given as
where EHOMO and ELUMO are the energies of the highest occupied and lowest unoccupied molecular orbitals, respectively. The electrophilicity index is defined as (Chattaraj et al., 2006)
3. RESULTS and DISCUSSION
Fast pyrolysis of WT and CAD mutant samples was conducted at 400 and 500 °C (Fig. 3). Table 1 shows the list of compounds analyzed from the pyrolysis runs. As presented, 22 lignin derivatives were identified and quantified. The total amount of lignin derivatives from the pyrolysis of WT was 57.3 and 91.8 μg/mg lignin at 400 and 500 °C, respectively. Among the compounds analyzed, guaiacol, creosol, eugenol, 4-vinylguaiacol and isoeugenol were the major products from WT. In CAD mutant lignin, the distribution of the pyrolysis products was different from WT and the total amount of the products was about 56.3 μg/mg lignin at 500 °C, which is 38.7% lower than WT. Syringol, guaiacol, p-cresol, toluene and creosol were the main products from the pyrolysis of CAD mutant. Interestingly, aromatic hydrocarbons including toluene, ethylbenzene and styrene were produced from the pyrolysis of CAD mutant whereas the yields of those from the WT were insignificant. The pyrolysis products were further analyzed based on their substructure to understand how the thermal behavior of CAD-downregulated lignin is different from the WT lignin.
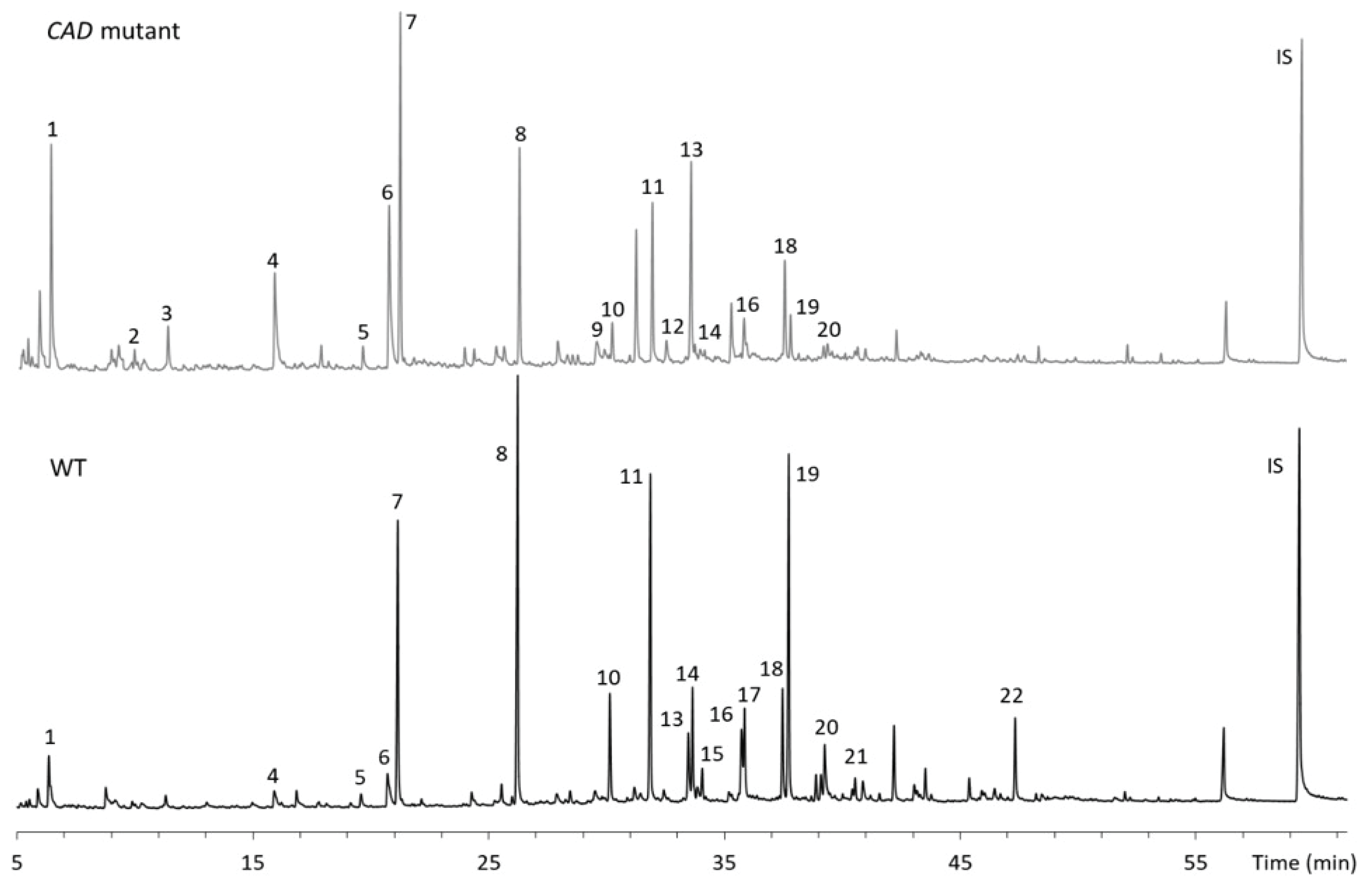
Fig. 4 shows the yield of pyrolysis products from lignins based on number of carbons in the side chain, namely C6, C1C6, C2C6 and C3C6. As shown in this figure, the yield of C6 units from CAD mutant was significantly higher that from WT. When pyrolyzed at 400 °C, the yield of C6 units from WT was 6.2 μg/mg lignin while that from CAD mutant was 17.0 μg/mg lignin. At 500 °C, the yield of C6 units from CAD transgenic further increased to 22.5 μg/mg lignin, which is almost a twofold compared to the WT. In contrast to the C6 products, the WT yielded higher yields of C1C6, C2C6 and C3C6 units at 400 and 500 °C. This implies that fragmentation of side chain in the lignin structure occurred significantly from the CAD mutant producing C6 product. Typically, thermal decomposition of lignin starts from the cleavage of aryl-ether linkage, the weakest bond in lignin structure, followed by a series of cleavage reactions. Apparently, the aldehyderich lignin from CAD mutant underwent secondary fragmentation reaction due to its reduced recalcitrance or increased reactivity.
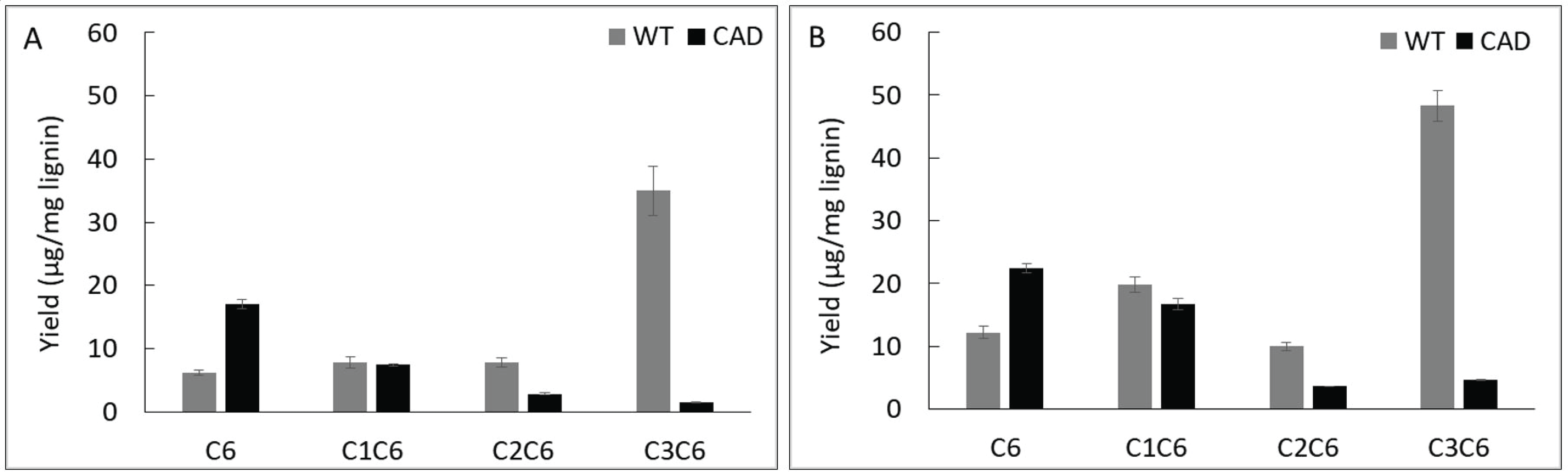
The pyrolysis products were further categorized by their subunits according to the number of methoxyl group (Fig. 5). At 400 °C, guaiacyl (G) units account for the majority of the pyrolysis products followed by syringyl (S) and p-hydroxy phenyl (H) units from the WT and transgenic lignin. At 500 °C, the same trend was observed from the WT whereas transgenic lignin yielded slightly higher H units (11.9 μg/mg lignin) than S units (11.0 μg/mg lignin) suggesting that defunctionalization reaction significantly occurred. This could be attributed to the increased reactivity of aldehyde-rich lignin structure upon pyrolysis at high temperature.
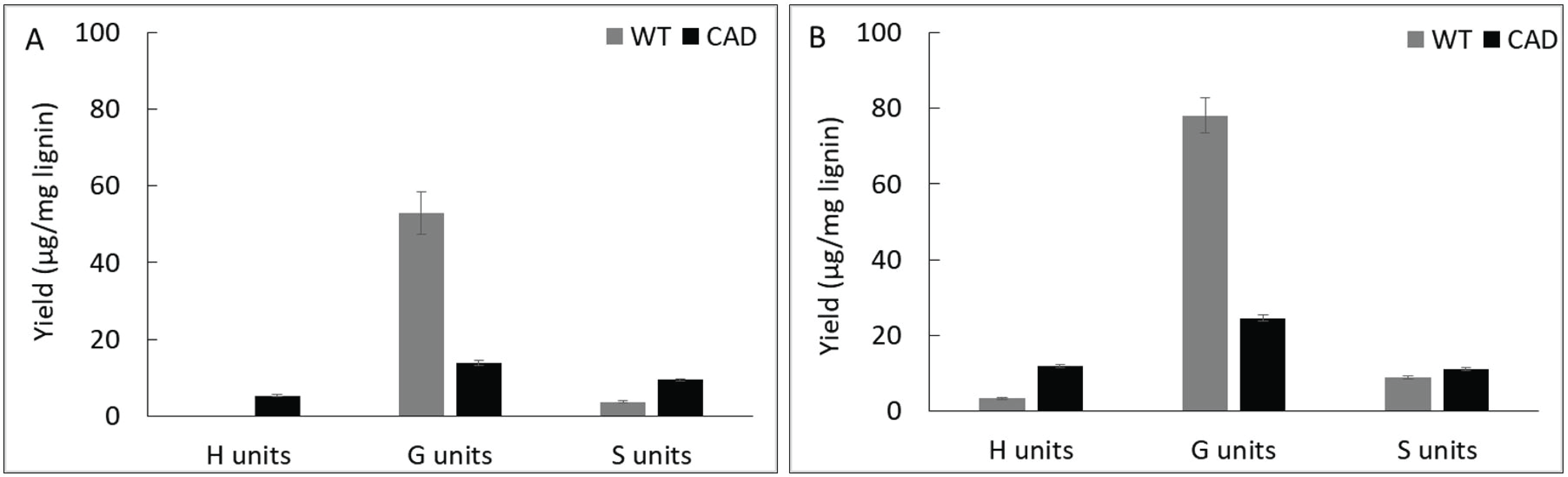
As observed above, aldehyde-rich lignin structure resulted from the strategic downregulation of CAD gene shows increased fragmentation reaction, which is different from the thermal behavior of WT lignin. Thus, it is logically hypothesized that CAD downregulated lignin has high reactivity toward chemical and/or thermochemical reactions. To prove this hypothesis, the computational simulation was performed to understand the effect of CAD downregulation on the chemical reactivity of lignin. In this work, each representative dimeric model compound from WT (β-O-4’) and CAD mutant (8-O-4’) was used for the computational study. Considering the complex and heterogeneous structure of lignin, it is reasonable to employ the lignin model dimers with representative interunit linkages (arylether). Fig. 6 illustrates the optimized geometry of two dimeric model compounds obtained from DFT based calculations. The electrophilicity index of two model dimers was calculated as a descriptor of reactivity that shows the global electrophilic nature of a molecule (Shi et al., 2016). As shown in Fig. 7, the electrophilicity index of a typical β-O-4’ dimer is 1.78 eV while that of 8-O-4’ dimer is 4.06 eV. Clearly, 8-O-4’ structure has a higher electrophilicity index, indicating that CAD mutant lignin is chemically more reactive compared to the WT lignin (Carmona et al., 2015). As observed from the pyrolysis, the relatively higher yield of fragmented products (i.e., C6 units) from CAD mutant is likely attributed to its reactive structure. Additionally, it should be noted that the higher reactivity of CAD mutant lignin could facilitate repolymerization and charring reaction. Aldehydes are reactive functional groups and typically responsible for repolymerization reaction during the depolymerization of lignin (Kim et al., 2015c). The lower yield of pyrolysis products from CAD mutant than WT is possibly associated with a highly reactive characteristic of aldehyde-rich lignin. Overall, the product distribution along with computational simulation indicate the occurrence of two competing reaction pathways, which are fragmentation and repolymerization reactions. Although more in-depth analyses are necessary to elucidate the reaction mechanisms, the results clearly support the hypothesis that CAD downregulation changes the thermal behavior and product distribution of lignin.
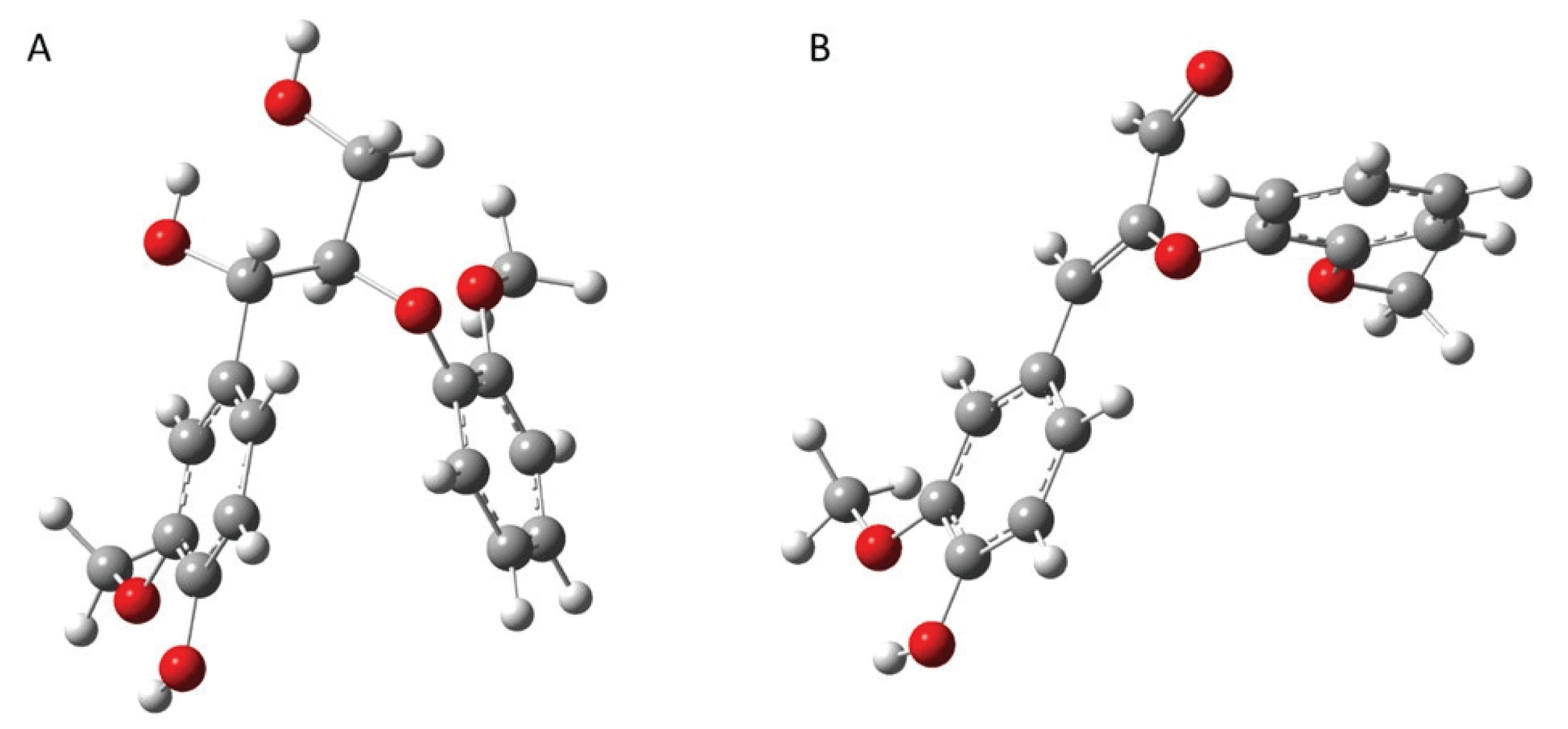
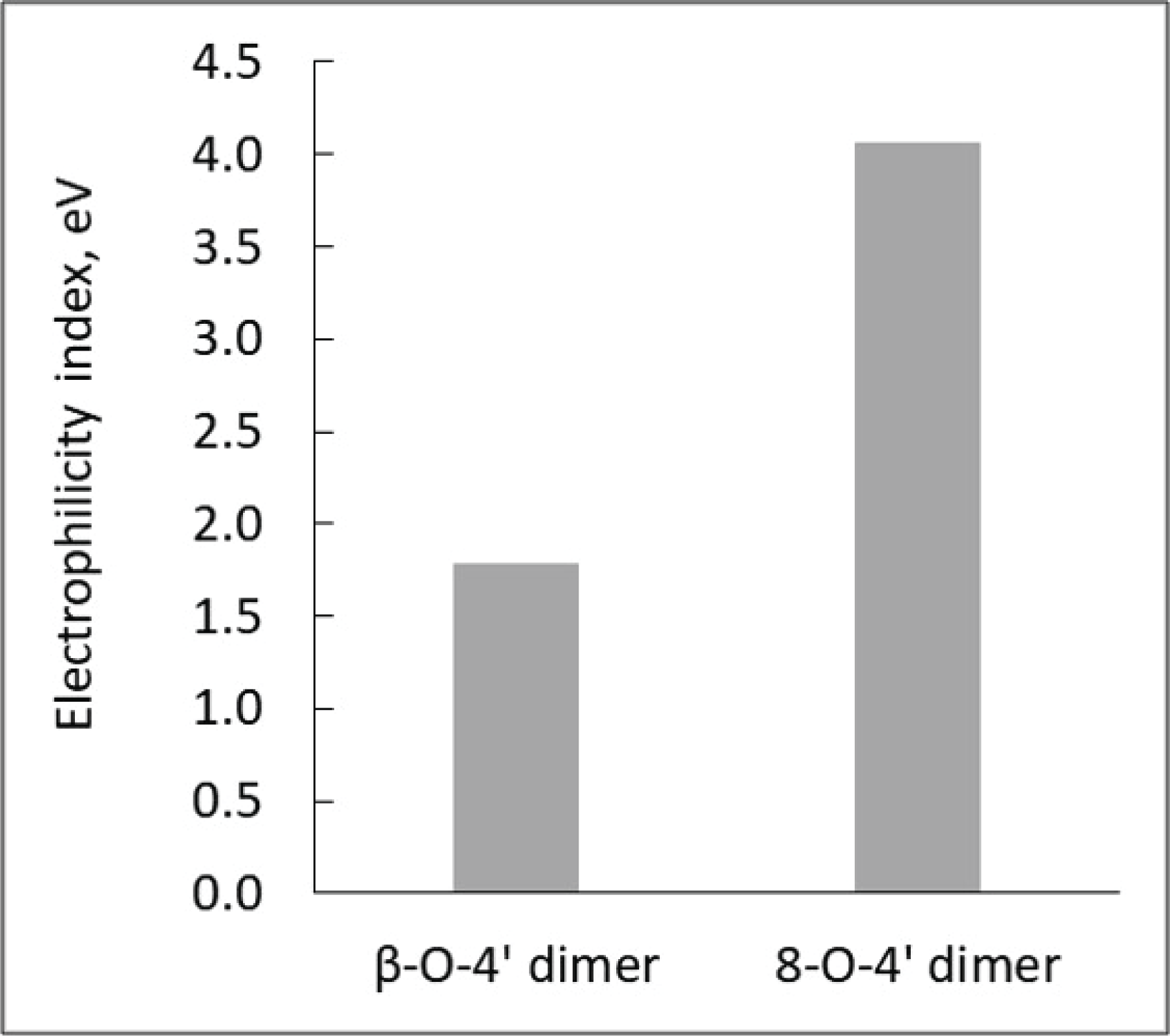
4. CONCLUSION
Plant genetic engineering has been widely used to overcome biomass recalcitrance. In this work, pyrolysis of lignins obtained from WT and CAD downregulated Arabidopsis was conducted to understand the effect of CAD downregulation on products distribution. The results suggest that CAD transgenic biomass undergoes relatively more fragmentation and repolymerization reaction compare to the WT. The highly reactive aldehyde-rich structure of CAD mutant, described by higher electrophilicity index calculated by computational analysis, clearly support the hypothesis. This result provides mechanistic insights toward biomass genetic engineering and its application to the thermal depolymerization. Finally, integrative and interdisciplinary research will provide the basis for establishing sustainable biorefinery in the future.