1. INTRODUCTION
Termites are wood-eating pests (Arinana et al., 2022; Su and Scheffrahn, 2000). Since termites damage wooden buildings, many studies have been conducted to control them in various ways (Adfa et al., 2020, 2023; Arsyad et al., 2020; Cahyono et al., 2020; Ella Nkogo et al., 2022; Im and Han, 2020; Lee et al., 2020; Oramahi et al., 2022; Priadi et al., 2023). However, they also serve as ecological suppliers providing various nutrients, including nitrogen compounds (Govorushko, 2019; Lobry de Bruyn and Conacher, 1990). They are widely distributed worldwide (Bourguignon et al., 2016), including Korea with freezing temperatures in winter (Cho et al., 2010b; Kim et al., 2012). In Korea, a representative lower termite species is Reticulitermes speratus (Cho et al., 2010b; Kim et al., 2012). It requires symbiotic microbes for the digestion of woody cellulose (Peterson and Scharf, 2016). Therefore, in the digestive tract of R. speratus, symbiotic microbes that aid in digestion must exist at low temperatures. In a previous study, a microorganism that produces β-glucosidase at 10°C was proposed among the symbiotic microorganisms in the gut of termites (Lee et al., 2018). In this study, we aim to show that the proposed low-temperature-produced β-glucosidases are not simply enzymes produced at low temperature, but that their enzymatic properties also have low-temperature enzymatic reaction characteristics.
Reticulitermes flavipes has been reported to survive at low temperatures. In the range of 8.1°C to 5.7°C, it enters a cold coma, and half of individuals have been reported to die (LT50) after 1 hour at –5.1°C (Clarke et al., 2013). Porotermes adamsoni and Stolotermesvictoriensis were active near 0°C, and half of individuals dying at –5.66°C and –7.32°C, respectively (Lacey et al., 2010). For R. speratus, half of the individuals died at –8.0°C, –8.0°C, –6.6°C, and –6.4°C after a 2-hour incubation of kings, queens, workers, and soldiers, respectively (Takata et al., 2023).
Cellulose, the main nutritional source found in wood, is efficiently broken down by three types of enzymes known as cellulases: endo-β-1,4-glucanase (EG), cellobiohydrolase (CBH), and β-glucosidase (Kumar et al., 2008). When termites crush cellulose, it undergoes degradation into smaller molecules through the action of EG, which is secreted by their salivary glands. In the case of the lower termite such as R. speratus, the cellulose is further converted into glucose with the additional CBH and β-glucosidase enzymes produced by symbiotic microorganisms in the termite’s gut. It has been suggested that R. speratus possesses three EG enzymes (Ahn and Kim, 2021), and certain symbiotic microorganisms also possess an EG enzyme (Otagiri et al., 2013). Significant reduction in cellulase activity within the hindgut was observed when bacteria were eliminated by ampicillin in Nasutitermes takasagoensi, indicating the involvement of symbiotic microorganisms in cellulose digestion (Tokuda and Watanabe, 2007). Symbiotic microbes found in termites produce CBH and β-glucosidase, but not EG, which are responsible for the initial breakdown of cellulose into smaller molecular weights (Cho et al., 2010a). This observation may be attributed to symbiotic adaptation, considering that the initial reduction in cellulose molecular weight occurs by EG produced in the termite’s mouth, and EG is not required for the subsequent stages of digestion. The population of the symbiotic bacterial community is conserved across different termites due to adaptations resulting from common dietary habits (Arora et al., 2022; Waidele et al., 2019).
The β-glucosidase production of bacteria isolated from the gut of R. speratus KMT1 shows various temperature specificities (Lee et al., 2018). In particular, Elizabethkingia miricola strain BM10 produces the maximum β-glucosidase activity at 10°C and exhibits a sharp decrease in β-glucosidase activity when the growth temperature is increased to 20°C. Therefore, E. miricola strain BM10 likely provides a decomposition mechanism of symbiotic microbes necessary for cellulose catabolism under low-temperature growth conditions of R. speratus KMT1.
In this study, the enzymatic characteristics of β-glucosidase produced by E. miricola strain BM10 at low temperature were analyzed. The psychrophilic β-glucosidase genes were identified by increased gene expression level by shifting the cell culture temperature from 30°C to 10°C. Two selected β-glucosidase genes were produced by heterologous expression in Escherichia coli and shown to be psychrophilic enzymes by characterizing the optimal reaction temperature.
2. MATERIALS and METHODS
E. miricola strain BM10 was isolated from the digestive tracts of R. speratus KMT1 (Lee et al., 2015).
The following media were prepared using the given components: tryptone yeast extract (TYE) medium with 1% tryptone (REF: 211705, Becton Dickinson, Seoul, Korea) and 0.5% yeast extract (REF: 212750, Becton Dickinson); yeast extract peptone (YEP) medium with 0.2% yeast extract and 0.8% peptone (REF: 211677, Becton Dickinson) in 0.1 M phosphate buffer (pH 7.0) made with sodium phosphate monobasic dihydrate (Product#: 1429, Duksan, Ansan, Korea) and sodium phosphate dibasic anhydrous (Product#: 1490, Duksan); Luria–Bertani medium with 1% LB broth (REF: 244620, Becton Dickinson); and lysis buffer with 50 mM NaH2PO4, 300 mM NaCl, and 10 mM imidazole. Agar plates were made with the corresponding medium and 1.5% Bacto agar (REF: 214010, Becton Dickinson). Carboxymethyl cellulose (CMC, Catalog number: R7257) was purchased from Sigma-Aldrich (Seoul, Korea).
E. miricola strain BM10 stored at –80°C was streaked on agar plates with the TYE medium, 1% CMC, and 1.5% Bacto agar. Cells were incubated at 26°C for 2 days. A single colony was inoculated in 5 mL of TYE medium with 1% CMC and incubated at 26°C for 2 days while shaking at 250 rpm. The precultured cells were inoculated into 100 mL of YEP medium with 2% CMC and cultured at 10°C for 3 days while shaking at 250 rpm. After 3 days in the main culture, 0.002% sodium azide was added, and the cells were removed by centrifugation with 12,000×g at 4°C for 10 min. The cell free supernatant of the main culture was used to measure the β-glucosidase activity at 30°C and pH 7 (Lee et al., 2018). The 20 μL of enzyme was mixed with 160 μL of 0.1 M potassium phosphate buffer (pH 7.0) and 20 μL of 10 mM ρ-nitrophenyl-β-D-glucopyranoside, incubated at 30°C for 30 min, and the reaction was stopped by mixing 20 μL of 2 M calcium carbonate. Absorbance was measured at a wavelength of 405 nm and the amount of ρ-nitrophenol produced was calculated using a ρ-nitrophenol standard curve. One unit of activity was defined as the amount of β-glucosidase activity that generated 1 μmol ρ-nitrophenol/min. The Cellic CTec2 (Novozymes A/S, Bagsværd, Denmark) was used as a positive control after 1/40,000 dilution. The Lineweaver–Burk equation was obtained using 1/[S] (1/mM) for χ value and 1/V (mL·min/μmol-ρ-nitrophenol) for y values.
The complete chromosomal DNA sequence of E. miricola strain BM10 is available at the National Center for Biotechnology Information (https://ncbi.nlm.nih.gov/; GenBank number: CP011059). The six proteins with β-glucosidase in the description were selected, and their closest proteins in the protein database were identified using protein BLAST.
E. miricola strain BM10 was cultured in accordance with the procedures described in the section “β-glucosidase activity of cell free culture broth”. Cells were harvested by centrifugation with 12,000×g at 4°C for 10 min. TRIzol® (1 mL; Catalog number: 15596026, Thermo Fisher Scientific, Seoul, Korea) was added and incubated at 4°C for 5 min. The cells were rapidly frozen and disrupted using TissueLyser LT (Qiagen, Seoul, Korea). After centrifugation at 12,000×g for 10 min, the supernatant was separated and mixed well with 200 μL of chloroform. The mixture was incubated at 23°C for 3 min. After another centrifugation at 12,000×g for 15 min, the supernatant was separated and mixed with 500 μL of isopropanol. The mixture was incubated at 23°C for 10 min, and the precipitated pellet was harvested by centrifugation at 12,000×g for 15 min. The pellet was rinsed with 1 mL of 75% cold ethanol. The extracted RNA was dried and dissolved in pure water treated with 0.1% (v/v) of diethyl pyrocarbonate. For cDNA synthesis, a mixture was made using M-MLV Reverse Transcriptase (Catalog number: M2410, GenDEPOT, Dawinbio, Hanam, Korea) in accordance with the manufacturer’s protocol. cDNA was synthesized at 42°C for 60 min, and the enzyme was inactivated by boiling for 10 min.
For RT-PCR, seven primer pairs of 6 β-glucosidase candidate genes and glyceraldehyde 3-phosphate dehydrogenase A gene as a reference gene of E. miricola strain BM10 were designed: 0821F (5’-GAAGGATTGG GACTGGGAAT-3’) and 0821R (CAAATTTCTGCACC AGCTCA) for bglX_1; 1398F (CTGGTTTCCGGTAAT GCTGT) and 1398R (CCATCTGATGAGCTGCGTTA) for bglB_1; 3284F (AGCCAAGATGGGGAAGAGTT) and 3284R (CTTCCGGTGCTCCATACAGT) for bglX_2; 3419F (ACCATCCAATAATCCGGTGA) and 3419R (TTTTCTGAGGGTTCCACAGG) for bglX_3; 3735F (GCCACTGCTTTTCCTGTAGC) and 3735R (CATTTT CCTGAACGCCATTT) for bglB_2; 3904F (CCCGGCA GAAAAAGTATTGA) and 3904R (CGGTTAAGCAAA AGGTCTGC) for bglA_2; and G3PDHF (CACGGGC AATTTAAAGGAGA) and G3PDHR (GCGTTAATGT GAGCTTGTGC) for gapA.
RT-PCR was performed with 10 μL of iQTM SYBR®Green Supermix (Catalog number: 1708880, Bio-Rad Laboratories, Hercules, CA, USA), 1 μL of forward primer, 1 μL of reverse primer, 1 μL of cDNA, and 7 μL of deionized water under the following reaction conditions: 1 cycle at 95°C for 5 min; 40 cycles at 95°C for 45 s, 55°C for 30 s, and 72°C for 30 s; and 1 cycle at 72°C for 10 min. The specificity of the RT-PCR products was confirmed through melting curve analysis between 60°C and 95°C. RT-PCR was performed thrice.
The expression plasmid was constructed and transformed into E. coli TOP 10 by Cosmogentech (Seoul, Korea). bglB_1 and bglA_2 were amplified through PCR. Since bglB_1 was difficult to amplify by a single PCR experiment, internal primers were used to amplify bglB_1 in two segments. Subsequently, the resulting PCR products were combined and used as the template for a subsequent PCR reaction to amplify the complete bglB_1 gene. The primers for bglB_1 were BGLB1-NdeIF (CGCGCGGCAGCCATATGCTGAAGAGAATT ATCTTAGCC), BGLB1-XhoIR (GGTGGTGGTGCTCGAGTTACTGAAGTGTAAACTTCAC), BGLB1-intP1 (GAATTCCGCCATACTAGC), and BGLB1-intP2 (TAAGATGATGACTGTTGG). The primers for bglA_2 were BGLA2-NdeIF (CGCGCGGCAGCCATATGTTA TTGACAAAGGAAGCC) and BGLA2-XhoIR (GGTGG TGGTGCTCGAGTTATTTAATAAAATCGGCATACC). The restriction enzyme sites of NdeI in BGLB1-NdeIF and BGLA2-NdeIF and XhoI in BGLB1-XhoIR and BGLA2-XhoIR were underlined. PCR was performed with 1 μL of cDNA template, 5 μL of the forward primer, 5 μL of the reverse primer, 1 μL of Pfu DNA polymerase, 4 μL of dNTP mixture, 10 μL of 5× reaction buffer, and 24 μL of distilled water under the following reaction conditions: 1 cycle at 95°C for 5 min; 35 cycles at 95°C for 60 s, 55°C for 60 s, and 72°C for 60 s per kilobase; and 1 cycle at 72°C for 7 min.
The vector, pET28a, and the PCR products were cut by NdeI and XhoI. The cut pET28a with 4 μL was mixed with 1 μL of the PCR-amplified gene, 1 μL of ligase, 1 μL of 10× reaction buffer, and 3 μL of distilled water. After incubation at 23°C for 150 s, the mixture was cooled down at 4°C for 10 min. The ligated recombinant pET28a was transformed into E. coli Top10 via the heat shock method at 42°C for 90 s. The successful transformant produced colonies on LB agar plates with 50 μg/mL kanamycin. The recombinant plasmid was confirmed through colony PCR.
pET28a with the inserted gene was transformed into E. coli BL21 strain via the heat shock method, and the transformed cells were spread on LB agar plates with 50 μg/mL kanamycin. After colony selection, single colonies were obtained by streaking on LB agar plates with 50 μg/mL kanamycin again. The cells were cultured in LB broth at 37°C overnight and stored with 25% glycerin at –80°C.
For protein production, the cells were streaked and grown on LB agar plates with 50 μg/mL kanamycin. A single colony was inoculated in 5 mL of LB medium test tubes and inoculated at 37°C overnight. The precultured cells was inoculated into 100 mL of LB broth and cultured at 17°C for 18 h. Protein expression was induced by 0.01 mM isopropyl β-D-1-thiogalactopyranoside (IPTG; Catalog number: I0355, GenDEPOT).
Cells were harvested by centrifugation at 4,000×g for 20 min. The cell pellet was resuspended in 5 mL of lysis buffer and mixed with 25 μg/mL lysozyme. After incubation for 30 min, the cells were disrupted through sonication. Cellular extracts were centrifuged at 10,000×g for 20 min to separate the supernatant (soluble protein) from the pellet (insoluble protein). For protein analysis, proteins were denatured at 95°C for 5 min and separated via sodium dodecyl sulfate-polyacrylamide gel electrophoresis with 10% polyacrylamide. Proteins were stained with Coomassie brilliant blue R (Sigma-Aldrich).
The β-glucosidase activity of heterologous expressed β-glucosidases was measured using the supernatant of cell-free extracts in accordance with previously described methods (Lee et al., 2018) with some modifications. For enzyme activity measurement, the conditions used other than the conditions to be determined in each experiment were 20°C and pH 7.
Temperature-related changes in β-glucosidase activity were measured in the range of 5°C to 45°C at 5°C intervals to evaluate the psychrophilic enzymatic characteristics. The effect of pH on the β-glucosidase activity was determined with 100 mM sodium citrate buffer (pH 3-6), 100 mM sodium phosphate buffer (pH 6-8), and 100 mM Tris-HCl buffer (pH 8-9). For the thermostability test, enzyme activities were examined at the indicated temperature after incubation for the indicated time in each experiment. Ion-induced changes in β-glucosidase activity were examined with 1 mM of CuSO4, MnCl2, CoCl2, MgCl2, BaCl2, KCl, FeSO4, ZnSO4, FeCl3, CaCl2, and NaCl in order to evaluate the inhibitory effect of ions (Singh et al., 2023; Yan and Lin, 1997).
3. RESULTS and DISCUSSION
In a previous study, the maximum activity of β-glucosidase from E. miricola strain BM10 was detected at 10°C, and less than 20% of β-glucosidase activity was observed at above 20°C compared with that at 10°C (Lee et al., 2018). Enzyme kinetic analysis was performed to reveal the enzymatic characteristics of β-glucosidase expressed at a low temperature (Fig. 1). Since the β-glucosidase activity of Cellic CTec2, a positive control, was too high, it was diluted 40,000 times to resemble the β-glucosidase activity of E. miricola strain BM10. Enzyme activity affects Vmax (the maximal reaction velocity when the enzyme is saturated with its substrate) but not Km (the substrate concentration at which half of the maximum reaction velocity is reached) because of the unique nature of the enzyme. Km is an index indicating the affinity of an enzyme to its substrate; the lower the Km, the higher the substrate affinity. E. miricola strain BM10 and diluted Cellic CTec2 had Km of 1.01 and 4.74 mM, respectively. The Km of β-glucosidase from Cellic CTec2 was 4.69 times higher than that of β-glucosidase from E. miricola strain BM10. This relatively low Km indicated that the β-glucosidase from E. miricola strain BM10 had a 4.69-fold higher substrate affinity than Cellic CTec2. Enzymes generally react more rapidly with increasing temperature until they are inactivated through denaturation. High substrate affinity is a way to increase the reaction rate of enzymes, allowing them to maintain their activity even at low temperatures. Fig. 1 suggested that the reaction rate of β-glucosidase from E. miricola strain BM10 could be maintained at low temperatures by increasing substrate affinity. Many β-glucosidases have been reported to have low Km (Bockwoldt and Ehrmann, 2022; DelPozo et al., 2012; Pyeon et al., 2019; Zhou et al., 2011), and their optimum reaction temperature is 35°C–55°C. Crude enzymes do not accurately represent the properties of specific enzymes. However, the high substrate affinity of the crude enzyme confirmed the existence of psychrophilic β-glucosidases, and it was necessary to identify psychrophilic β-glucosidases to support the low-temperature habitat of R. speratus KMT1.
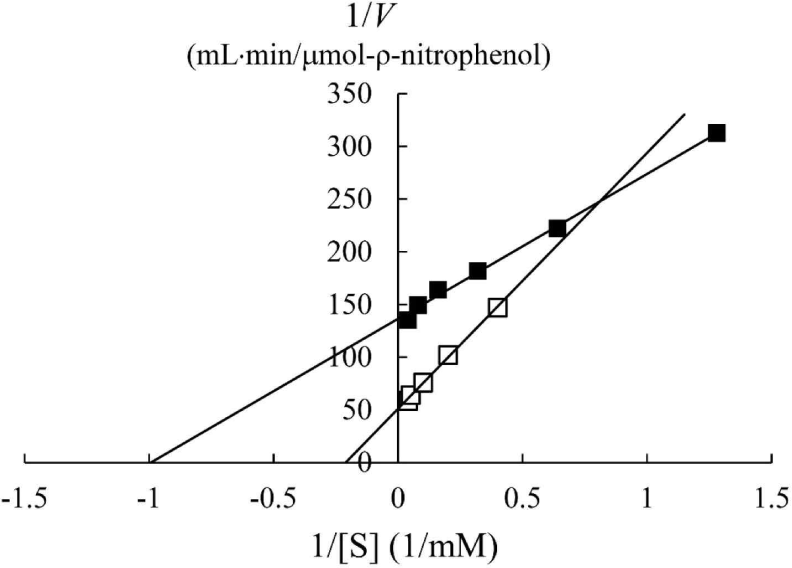
The complete chromosomal sequence of E. miricola strain BM10 was registered with accession number CP011059 at the National Center for Biotechnology Information (Lee et al., 2015). Six β-glucosidase genes were found in the entire chromosome of E. miricola strain BM10 (Table 1). The most homologous non-Elizabethkingia proteins, along with the names and amino acid numbers of the six genes, were displayed in the protein database. The percent identity of the six genes with the most homologous protein ranged from 67% to 85%. This low percent identity range with the closest proteins suggested that the β-glucosidases from E. miricola strain BM10 may have unique enzymatic characteristics that differ from those of other currently reported species.
Since E. miricola strain BM10 showed a maximum β-glucosidase activity when grown at 10°C (Lee et al., 2018), β-glucosidase would be expressed at 10°C. The expression levels of the genes proposed as β-glucosidase at 10°C and 30°C were compared through RT-PCR (Fig. 2). The genes whose expression levels were more than doubled at 10°C than at 30°C were bglB_1 (6.98-fold) and bglA_2 (2.28-fold). These results suggested that bglB_1 and bglA_2 were responsible for the high β-glucosidase activity at 10°C in E. miricola strain BM10.
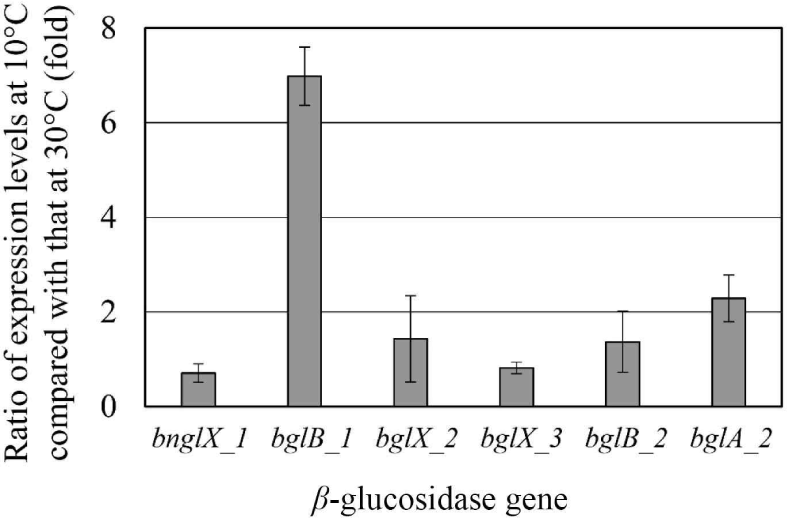
bglB_1 and bglA_2 were independently inserted into the pET28a vector, and the recombinant plasmids were obtained from E. coli TOP10. The inserted gene in the recombinant plasmids was confirmed through sequencing analysis (data not shown). The confirmed recombinant plasmids were introduced in the expression strain E. coli BL21. The expression of both genes was induced with 0.01 mM IPTG at 17°C for 18 h. Large amounts of proteins with the desired size were produced in the cells by IPTG for each protein compared with those in the cells without the expressed genes (Fig. 3). Most of the produced proteins were observed in the inclusion bodies, and only a small amount of the protein was produced in a soluble state.
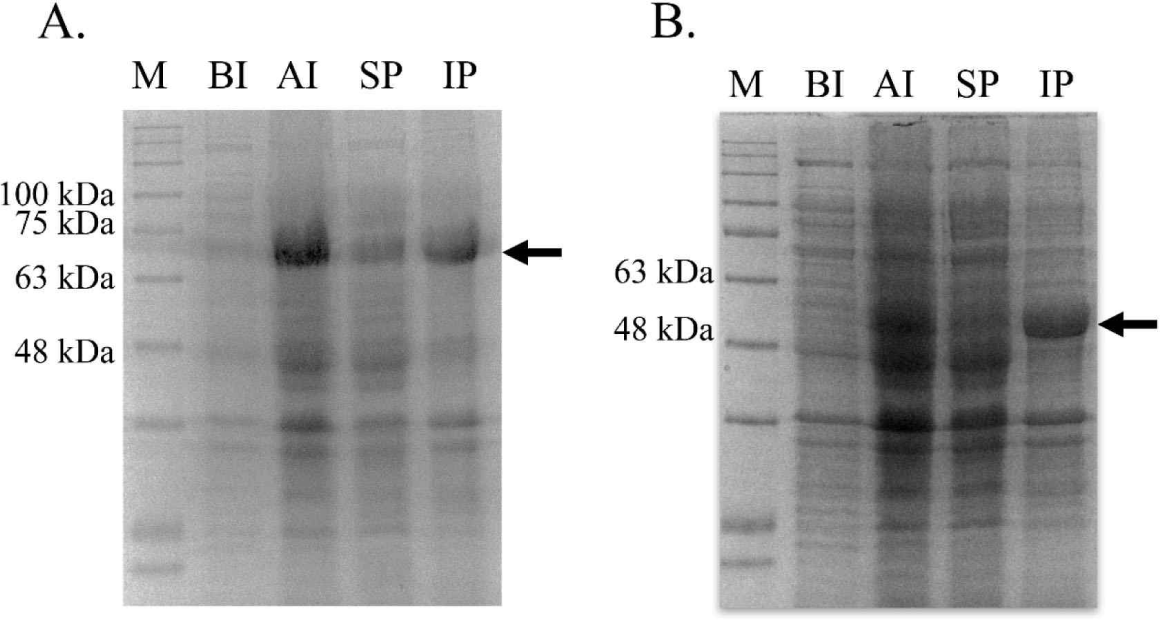
After the proteins were produced, their β-glucosidase activities were measured using the soluble fraction. The β-glucosidase activities of AJW62880 (the protein of bglB_1) and AJW65329 (the protein of bglA_2) at 17°C were 1.582 ± 0.013 and 0.350 ± 0.011 U/mL, respectively. The β-glucosidase activities were 7.5-fold higher for bglB_1-expressing cells and 1.6-fold higher for bglA_2-expressing cells than those of 0.210 U/mL in the cell-free culture medium of E. miricola strain BM10. Although many expressed proteins were insoluble, a sufficient enzyme activity was observed in soluble proteins to characterize the enzymes.
The enzymatic characteristics of β-glucosidase were evaluated with the heterologous expressed soluble enzyme. In the case of AJW62880, the optimum reaction temperature and pH were 20°C (Fig. 4A) and 6.5 (Fig. 4B), respectively. In the thermostability test (Fig. 4C), most of the β-glucosidase activity remained at 30°C for 2 h, but the enzyme activity rapidly disappeared within 15 min at 40°C. No significant ion-induced changes in enzyme activity were observed (Fig. 4D). Even when the enzyme activity was slightly reduced by some ions, the enzyme activity was not significantly changed, considering that the enzyme activity was not changed by the same ion provided as a different type of salt.
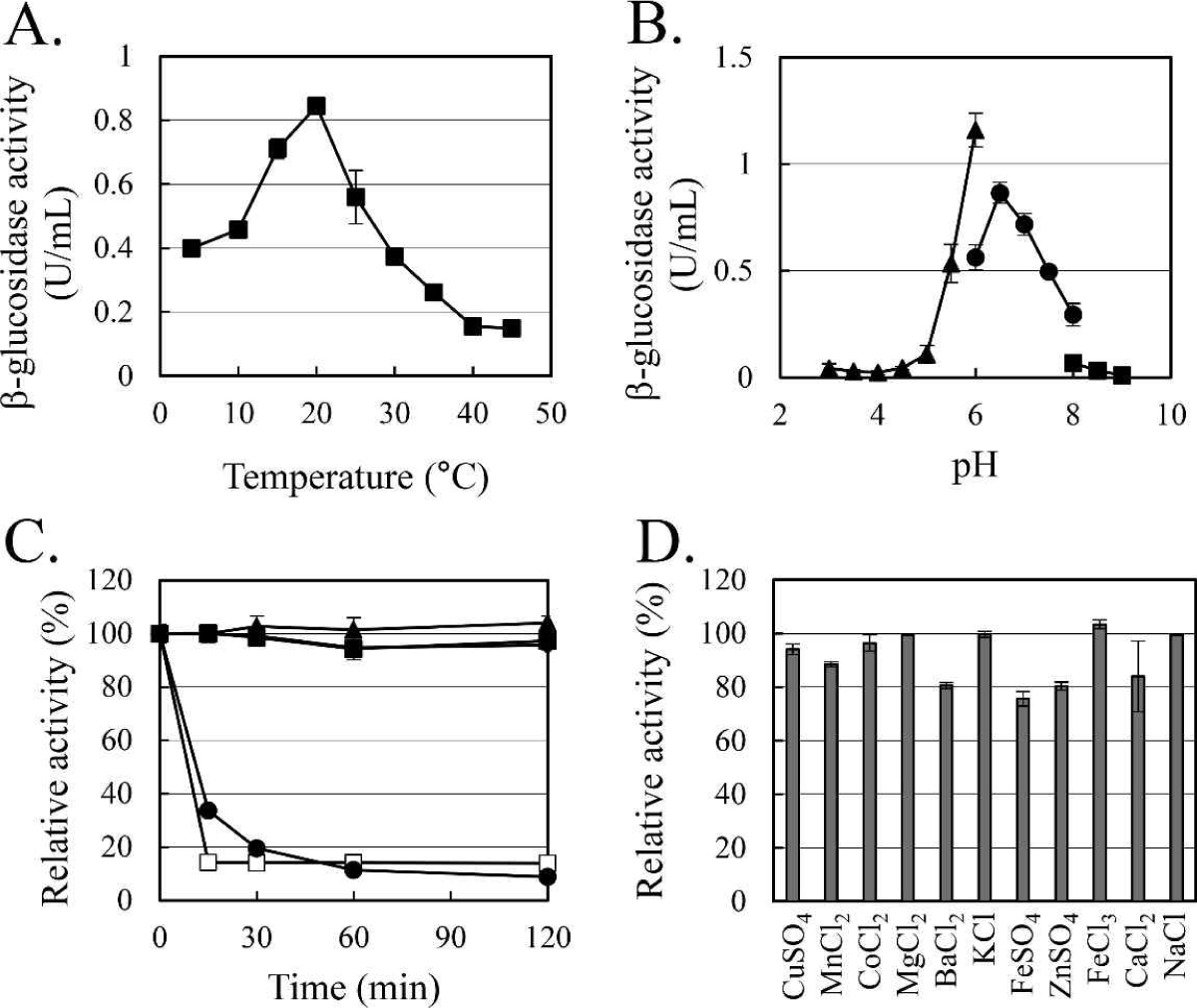
In the case of AJW65329, the optimum reaction temperature and pH were 25°C (Fig. 5A) and 7.0 (Fig. 5B), respectively. In the thermostability test (Fig. 5C), similar to that observed in AJW62880, β-glucosidase activity was mostly maintained at 30°C for 2 h. However, at 40°C, the enzyme activity of AJW65329 disappeared more slowly than that of AJW62880. The β-glucosidase activity of AJW65329 was severely affected by ions (Fig. 5D). Particularly, CuSO4, FeSO4, and ZnSO4 strongly inhibited the enzyme activity by more than 60%.
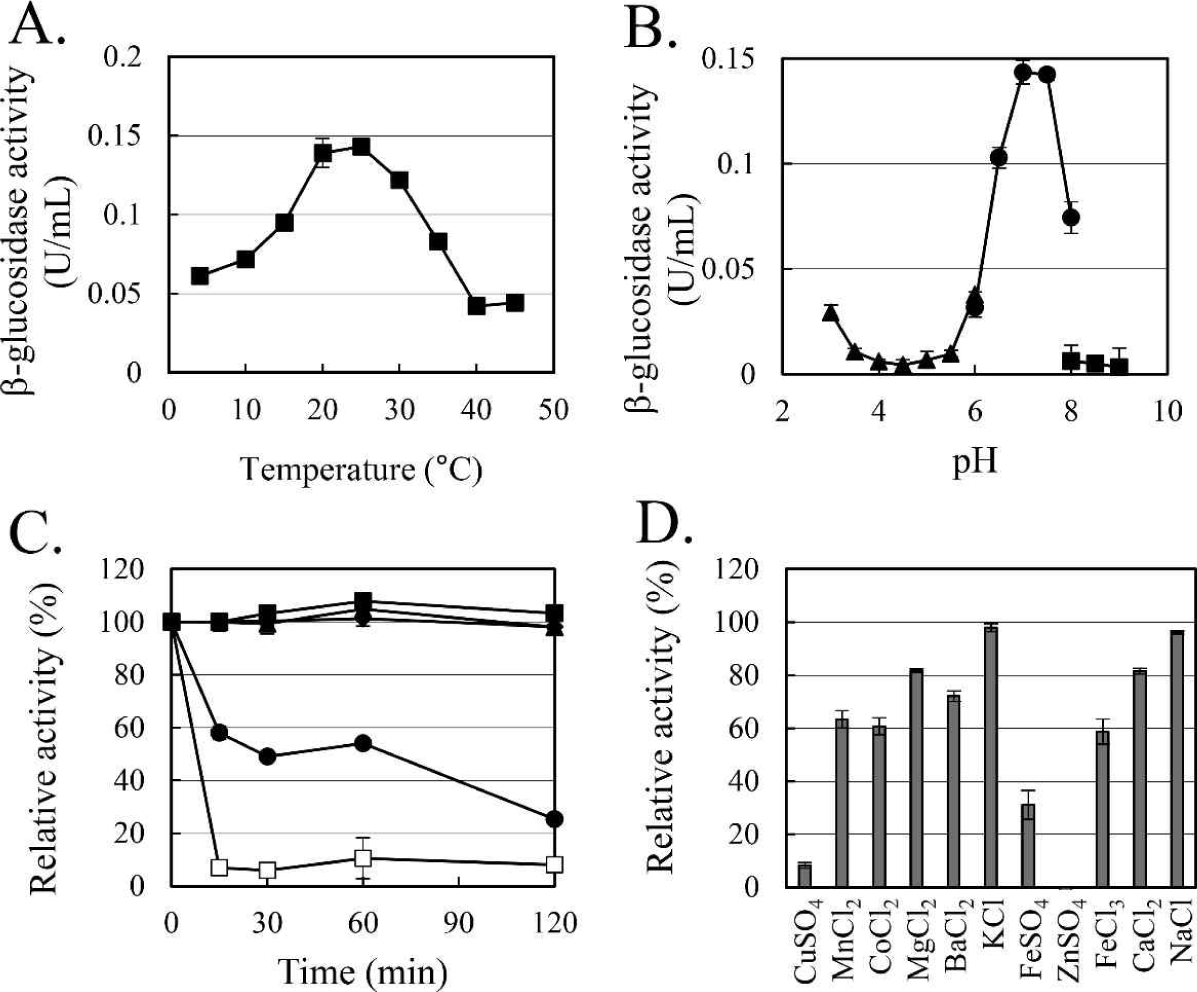
The enzymatic kinetics of two heterologous expressed β-glucosidases were analyzed (Fig. 6). AJW62880 and AJW65329 had Km of 48.53 and 17.92 mM, respectively. The substrate affinities of these two β-glucosidases were approximately 10 and 4 times lower than that of Cellic CTec2, which had Km of 4.74. This result is contrary to the high substrate affinity of β-glucosidase in the cell culture broth. This contrary Km value suggested that the enzyme structure was partially modified according to heterologous expression; thus, enzymatic characteristics changed, or a specific substance present in the cell culture broth increased the substrate affinity of β-glucosidase.
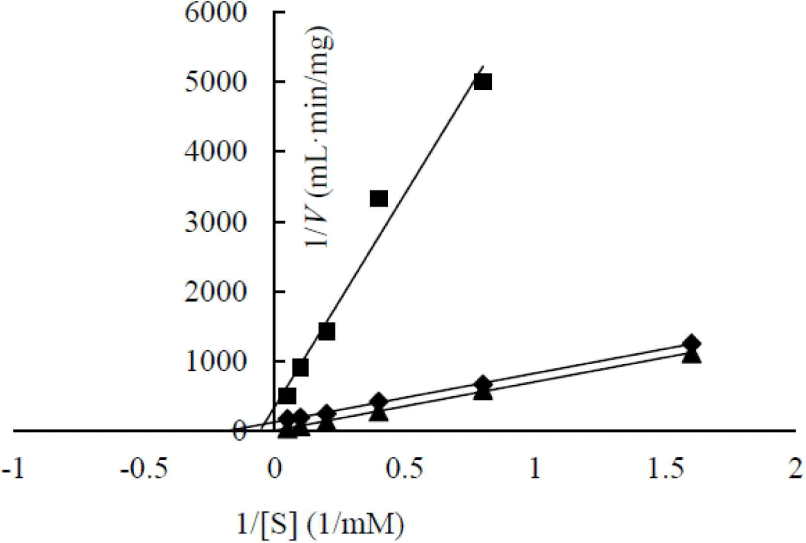
In a previous study, a low-temperature-active β-glucosidase was obtained from symbiotic Serratia sp. TN49 isolated from the gut of longhorned beetle (Batocera horsfieldi) larvae (Zhou et al., 2011), and its optimum reaction temperature is 35°C. In the present study, the optimal reaction temperature of two heterologous expressed β-glucosidases was 20°C–25°C, and the expression of E. miricola strain BM10 psychrophilic β-glucosidases at 10°C (Lee et al., 2018) suggested that AJW62880 and AJW65329 were β-glucosidases more optimized for low temperatures. R. speratus KMT1 feeds on wood and requires the help of commensal microorganisms in the gut to digest wood. To survive in regions with sub-zero temperatures in winter, R. speratus KMT1 needs symbiotic microorganisms that help in digestion in the intestines. Therefore, the results of this study provided an ecological basis for the spread of termite habitats in low-temperature regions.
Further studies may reveal the structural features of psychrophilic β-glucosidases with a high substrate affinity and identify the regulatory mechanism of cold gene expression specifically at 10°C.
4. CONCLUSIONS
E. miricola strain BM10, a termite-symbiotic bacterium, produced β-glucosidase with high substrate affinity at 10°C to have an efficient enzymatic reaction even at low temperatures. Two β-glucosidase genes, bglB_1 and bglA_2, expressed by E. miricola strain BM10 at low temperatures were identified. The heterologous expression of the identified genes in Escherichia coli confirmed that they were psychrophilic β-glucosidases with an optimal reaction temperature of 20°C–25°C. These results demonstrated that symbiotic microbes provided low-temperature-specific enzymes necessary for the survival of termites at low temperatures. Such symbiotic microbes allow termites to expand their habitats to low-temperature regions.